50 7.5 Currents, Upwelling and Downwelling
The movement of surface currents also plays a role in the vertical movements of deeper water, mixing the upper water column. Upwelling is the process that brings deeper water to the surface, and its major significance is that it brings nutrient-rich deep water to the nutrient-deprived surface, stimulating primary production (see section 8.3). Downwelling is where surface water is forced downwards, where it may deliver oxygen to deeper water. Downwelling leads to reduced productivity, as it extends the depth of the nutrient-limited layer.
Upwelling occurs where surface currents are diverging, or moving away from each other. As the surface waters diverge, deeper water must be brought to the surface to replace it, creating upwelling zones. The upwelled water is cold and rich in nutrients, leading to high productivity. Many of the most productive regions on Earth are found in upwelling zones. In the equatorial Pacific, the trade winds blow the North and South Equatorial Currents towards the west, while Ekman transport causes the upper layers to move to the north and south in their respective hemispheres. This creates a divergence zone, and a region of upwelling and high productivity (Figure 7.5.1).
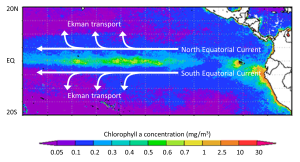
A similar process occurs near the Antarctic continent, creating one of the most productive regions on Earth, the Antarctic divergence. In this case, the West Wind Drift (Antarctic Circumpolar Current) is flowing parallel to, but in the opposite direction of the East Wind Drift. With both currents occurring in the Southern Hemisphere, Ekman transport will be to the left, so the eastward-flowing West Wind Drift water will be transported to the north, and the westward-flowing East Wind Drift water will be transported to the south, creating a highly productive divergence zone (Figure 7.5.2).
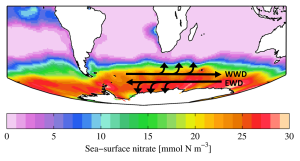
Downwelling occurs where surface currents converge. The converging water has nowhere to go but down, so the surface water sinks. Since surface water is usually low in nutrients, downwelling leads to low productivity zones. An example of a downwelling region is off of the Labrador coast in Canada, where the Gulf Stream, Labrador, and East Greenland Currents converge.
Coastal Upwelling
Upwelling and downwelling also occur along coasts, when winds move water towards or away from the coastline. Surface water moving away from land leads to upwelling, while downwelling occurs when surface water moves towards the land. Historically, some of the most productive commercial fishing grounds have been associated with coastal upwelling. Along the coast of California, the local prevailing winds blow towards the south. Ekman transport moves the surface layer 90o to the right of the wind, meaning the net Ekman transport is in an offshore direction. The water displaced near the coast is replaced by cold, nutrient-rich deeper water that is brought to the surface through upwelling, leading to high productivity (Figure 7.5.3).
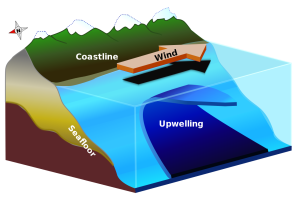
The same process happens off of the coast of Peru, which for a long time had the world’s largest commercial fishery. Winds along the Peruvian coast blow towards the north, and since Peru is in the Southern Hemisphere, the Ekman transport is 90o to the left of the wind, which causes the surface water to move offshore and leads to upwelling and productivity. In any coastal upwelling location, if the winds reverse, surface water moves towards the shore and downwelling is the result.
Upwelling can also occur due to geological features of the ocean floor. For example, as deep water currents encounter seamounts or other raised features, the water is forced upwards, bringing nutrient-rich water to the surface. This helps explain why productivity is often high in the water over seamounts.
It may seem odd to be discussing coral reefs in a section about geology, but due to the stony calcium carbonate skeletons secreted by many coral species, coral reefs are as interesting as geological features as they are biological ones. Corals grow best in warm, clear, tropical water, that is close enough to the surface for light to support photosynthesis by the algae living in the coral tissues. Because of this need for light, new coral will often grown on top of the stony skeletons of older corals.
In the 1830s Charles Darwin made some observations about different types of coral reefs, and hypothesized that they represent a progression from one form to the next. The types of reefs he examined were fringing reefs, barrier reefs, and atolls, which are associated with oceanic islands (Figure 2.10.1). Fringing reefs are reefs that are close to or are connected to shore. Barrier reefs are offshore reefs that are separated from the land by an expanse of water, such as a lagoon. Atolls are circular or oval reefs surrounding a lagoon, without any central land mass in the lagoon. Darwin speculated that reefs progressed from fringing, to barrier, to atolls as the land mass subsided. However, he had no explanation for how volcanic islands could sink. Today we know that Darwin was correct, and that islands can sink as oceanic crust subsides as it moves away from a spreading center, or as sea level rises as glaciers melt.
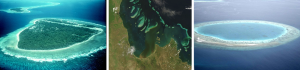
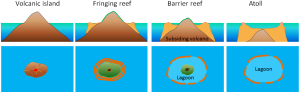
In the previous section we learned that rising air creates low pressure systems, and sinking air creates high pressure. In addition to their role in creating the surface winds, these high and low pressure systems also influence other climatic phenomena. Along the equator air is rising as it is warmed by solar radiation (section 6.2). Warm air contains more water vapor than cold air, which is why we experience humidity during the summer and not during the winter. The water content of air roughly doubles with every 10o C increase in temperature. So the air rising at the equator is warm and full of water vapor; as it rises into the upper atmosphere it cools, and the cool air can no longer hold as much water vapor, so the water condenses and forms rain. Therefore, low pressure systems are associated with precipitation, and we see wet habitats like tropical rainforests near the equator (Figure 6.3.1).
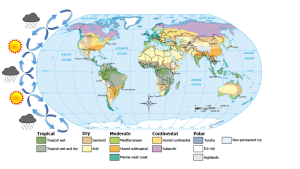
After rising and producing rain near the equator, the air masses move towards 30o latitude and sink back towards Earth as part of the Hadley convection cells. This air has lost most of its moisture after producing the equatorial rains, so the sinking air is dry, resulting in arid climates near 30o latitude in both hemispheres. Many of the major desert regions on Earth are located near 30o latitude, including much of Australia, the Middle East, and the Sahara Desert of Africa (Figure 6.3.1). The air also becomes compressed and heats up as it sinks, absorbing any moisture from the clouds and creating clear skies. Thus high pressure systems are associated with dry weather and clear skies. This cycle of high and low pressure regions continues with the Ferrel and Polar convection cells, leading to rain and the boreal forests at 60o latitude in the Northern Hemisphere (there are no corresponding large land masses at these latitudes in the Southern Hemisphere). At the poles, descending, dry air produces little precipitation, leading to the polar desert climate.
The elevation of the land also plays a role in precipitation and climactic characteristics. As moist air moves over land and encounters mountains it rises, expands, and cools because of the declining pressure and temperature. The cool air holds less water vapor, so condensation occurs and rain falls on the windward side of the mountains. As the air passes over the mountains to the leeward side, it is now dry air, and as it sinks the pressure increases, it heats back up, any moisture revaporizes, and it creates dry, deserts regions behind the mountains (Figure 6.3.2). This phenomenon is referred to as a rain shadow, and can be found in areas such as the Tibetan Plateau and Gobi Desert behind the Himalayas, Death Valley behind the Sierra Nevada mountains, and the dry San Joaquin Valley in California.
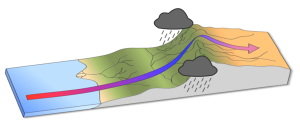
Rising and falling air are also responsible for more localized, short-term wind patterns in coastal areas. Due to the high heat capacity of water, land heats up and cools down about five times faster than water. During the day the sun heats up the land faster than it heats the water, setting up a convection cell of warmer rising air over the land and sinking cooler air over the water. This creates winds blowing from the water towards the land during the day and early evening; a sea breeze (Figure 6.3.3). The opposite occurs at night, when the land cools more quickly than the ocean. Now the ocean is warmer than the land, so air rises over the water and sinks over the land, creating a convection cell where winds blow from land towards the water. This is a land breeze, which blows at night and into the early morning (Figure 6.3.3).
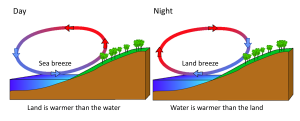
The same phenomenon leads to seasonal climatic changes in many areas. During the winter the lower pressure is over the warmer ocean, and the high pressure is over the colder land, so winds blow from land to sea. In summer the land is warmer than the ocean, causing low pressure over the land and winds to blow from the ocean towards the land. The winds blowing from the ocean contain a lot of water vapor, and as the moist air passes over land and rises, it cools and condenses causing seasonal rains, such as the summer monsoons of southeast Asia (Figure 6.3.4).

Learning Objectives
After reading this chapter you should be able to:
- identify the parts of a basic wave
- define the terminology used to describe the motion of a wave (i.e. period, frequency, speed etc.)
- explain the circular motion of water particles involved in wave motion
- explain the difference between deep water waves and shallow water waves
- identify the factors that influence wave speed in deep and shallow waves
- identify the factors that determine the energy of wind-generated waves
- define the concept of restoring force
- define significant wave height
- explain the creation of ocean swell
- define the concepts of destructive, constructive and mixed interference
- explain why waves break as they approach shore
- explain the differences in the different types of breakers, and how the bottom topography impacts breaker type
- explain why waves approach parallel to shore, and why waves are larger off of points and smaller in bays
- explain how tsunamis are formed, and how they behave in the ocean
- explain Newton's Law of Universal Gravitation and how it applies to tides
- explain why most places on Earth experience two tides per day, not just the one predicted from gravitational attraction between the Earth and moon
- explain how the Earth, sun and moon interact to create spring and neap tides
- explain why the sun has a smaller effect on tides than the moon
- explain why tides do not occur at the same time every day
- explain the concept of amphidromic circulation
- identify diurnal, semi-diurnal, and mixed tides
- identify the phases of a tidal current
- define a tidal bore
Waves come in many shapes and sizes; a 100 foot wave might be a surfer's dream, but a ship captain's nightmare. What was the largest wave ever recorded? 50 feet? 100 feet? Not even close. That record belongs to a wave created in Lituya Bay, Alaska, on July 9, 1958 (Figure 3.1). On that day, a magnitude 7.8 earthquake caused a massive rockslide that slid down a mountainside and into the headwaters of the bay. The rockslide created a splash wave that was high enough to flatten vegetation up to 1722 ft (525 m) above sea level! The wave then moved through the narrow bay towards the sea, destroying a number of fishing boats along the way. Miraculously, a father and son on one fishing boat were carried above the trees by the wave, and survived to tell the story. This is by far the largest wave, a megatsunami, ever reliably recorded. The waves we will discuss in this chapter may not be quite that dramatic, but it is still important to know how they form, how they are propagated, and what happens to them as they interact with the shore.
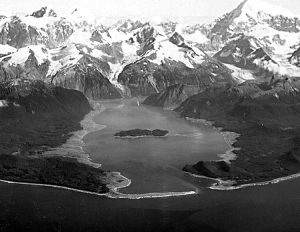
After discussing various types of waves at sea and along the shore, we will discuss tides. However, at least in terms of wavelength, the largest waves in the ocean are the tides, where one wavelength stretches halfway around the Earth. The crests of these long waves represent the high tides, while the troughs create low tides.
You probably learned when you were younger that the basic cause of the tides is the gravitational attraction between the Earth and moon. This is a very old idea, as the Greek scientist Pytheas first made the connection between the tides and the moon back in 330 B.C.E. Isaac Newton's gravitational work the 1600s led to our modern understanding of tidal cycles, however, we now know that the tides involve a lot more than just the Earth and the moon. There are many variables that influence the tides, yet despite this complexity, we are able to create accurate tide charts predicting the heights and timing of tides months or even years in advance.
Modified from Paul Webb, used under a CC-BY 4.0 international license. Download this book for free at https://rwu.pressbooks.pub/webboceanography/front-matter/preface/
Oxygen and carbon dioxide are involved in the same biological processes in the ocean, but in opposite ways; photosynthesis consumes CO2 and produces O2, while respiration and decomposition consume O2 and produce CO2. Therefore it should not be surprising that oceanic CO2 profiles are essentially the opposite of dissolved oxygen profiles (Figure 5.5.1). At the surface, photosynthesis consumes CO2 so CO2 levels remain relatively low. In addition, organisms that utilize carbonate in their shells are common near the surface, further reducing the amount of dissolved CO2.
In deeper water, CO2 concentration increases as respiration exceeds photosynthesis, and decomposition of organic matter adds additional CO2 to the water. As with oxygen, there is often more CO2 at depth because cold bottom water holds more dissolved gases, and high pressures increase solubility. Deep water in the Pacific contains more CO2 than the Atlantic as the Pacific water is older and has accumulated more CO2 from the respiration of benthic organisms.
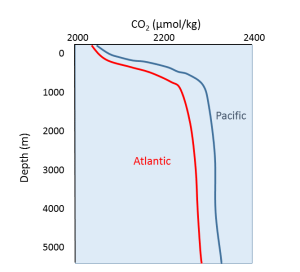
But the behavior of carbon dioxide in the ocean is more complex than the figure above would suggest. When CO2 gas dissolves in the ocean, it interacts with the water to produce a number of different compounds according to the reaction below:
CO2 + H2O ↔ H2CO3 ↔ H+ + HCO3- ↔ 2H+ + CO32-
CO2 reacts with water to produce carbonic acid (H2CO3), which then dissociates into bicarbonate (HCO3-) and hydrogen ions (H+). The bicarbonate ions can further dissociate into carbonate (CO32-) and additional hydrogen ions (Figure 5.5.2).

Most of the CO2 dissolving or produced in the ocean is quickly converted to bicarbonate. Bicarbonate accounts for about 92% of the CO2 dissolved in the ocean, and carbonate represents around 7%, so only about 1% remains as CO2, and little gets absorbed back into the air. The rapid conversion of CO2 into other forms prevents it from reaching equilibrium with the atmosphere, and in this way, water can hold 50-60 times as much CO2 and its derivatives as the air.
CO2 and pH
The equation above also illustrates carbon dioxide's role as a buffer, regulating the pH of the ocean. Recall that pH reflects the acidity or basicity of a solution. The pH scale runs from 0-14, with 0 indicating a very strong acid, and 14 representing highly basic conditions. A solution with a pH of 7 is considered neutral, as is the case for pure water. The pH value is calculated as the negative logarithm of the hydrogen ion concentration according to the equation:
pH = -log10[H+]
Therefore, a high concentration of H+ ions leads to a low pH and acidic condition, while a low H+ concentration indicates a high pH and basic conditions. It should also be noted that pH is described on a logarithmic scale, so every one point change on the pH scale actually represents an order of magnitude (10 x) change in solution strength. So a pH of 6 is 10 times more acidic than a pH of 7, and a pH of 5 is 100 times (10 x 10) more acidic than a pH of 7.
Carbon dioxide and the other carbon compounds listed above play an important role in buffering the pH of the ocean. Currently, the average pH for the global ocean is about 8.1, meaning seawater is slightly basic. Because most of the inorganic carbon dissolved in the ocean exists in the form of bicarbonate, bicarbonate can respond to disturbances in pH by releasing or incorporating hydrogen ions into the various carbon compounds. If pH rises (low [H+]), bicarbonate may dissociate into carbonate, and release more H+ ions, thus lowering pH. Conversely, if pH gets too low (high [H+]), bicarbonate and carbonate may incorporate some of those H+ ions and produce bicarbonate, carbonic acid, or CO2 to remove H+ ions and raise the pH. By shuttling H+ ions back and forth between the various compounds in this equation, the pH of the ocean is regulated and conditions remain favorable for life.
CO2 and Ocean Acidification
In recent years there has been rising concern about the phenomenon of ocean acidification. As described in the processes above, the addition of CO2 to seawater lowers the pH of the water. As anthropogenic sources of atmospheric CO2 have increased since the Industrial Revolution, the oceans have been absorbing an increasing amount of CO2, and researchers have documented a decline in ocean pH from about 8.2 to 8.1 in the last century. This may not appear to be much of a change, but remember that since pH is on a logarithmic scale, this decline represents a 30% increase in acidity. It should be noted that even at a pH of 8.1 the ocean is not actually acidic; the term "acidification" refers to the fact that the pH is becoming lower, i.e. the water is moving towards more acidic conditions.
Figure 5.5.3 presents data from observation stations in and around the Hawaiian Islands. As atmospheric levels of CO2 have increased, the CO2 content of the ocean water has also increased, leading to a reduction in seawater pH. Some models suggest that at the current rate of CO2 addition to the atmosphere, by 2100 ocean pH may be further reduced to around 7.8, which would represent more than a 120% increase in ocean acidity since the Industrial Revolution.

Why is this important? Declining pH can impact many biological systems. Of particular concern are organisms that secrete calcium carbonate shells or skeletons, such as corals, shellfish, and may planktonic organisms. At lower pH levels, calcium carbonate dissolves, eroding the shells and skeletons of these organisms (Figure 5.5.4).

Not only does a declining pH lead to increased rates of dissolution of calcium carbonate, it also diminishes the amount of free carbonate ions in the water. The relative proportions of the different carbon compounds in seawater is dependent on pH (Figure 5.5.6). As pH declines, the amount of carbonate declines, so there is less available for organisms to incorporate into their shells and skeletons. So ocean acidification both dissolves existing shells and makes it harder for shell formation to occur.

Additional links for more information:
- NOAA Ocean Acidification Program website http://oceanacidification.noaa.gov/