47 7.2 The Gulf Stream
The primary surface current along the east coast of the United States is the Gulf Stream, which was first mapped by Benjamin Franklin in the 18th century (Figure 7.2.1). As a strong, fast current, it reduced the sailing time for ships traveling from the United States back to Europe, so sailors would use thermometers to locate its warm water and stay within the current.
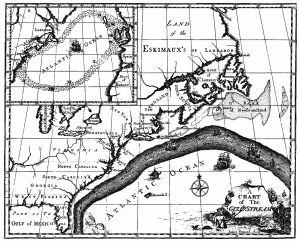
The Gulf Stream is formed from the convergence of the North Atlantic Equatorial Current bringing tropical water from the east, and the Florida Current that brings warm water from the Gulf of Mexico. The Gulf Stream takes this warm water and transports it northwards along the U.S. east coast (Figure 7.2.2). As a western boundary current, the Gulf Stream experiences western intensification (section 7.4), making the current narrow (50-100 km wide), deep (to depths of 1.5 km) and fast. With an average speed of 6.4 km/hr, and a maximum speed of about 9 km/hr, it is the fastest current in the world ocean. It also transports huge amounts of water, more than 100 times greater than the combined flow of all of the rivers on Earth.
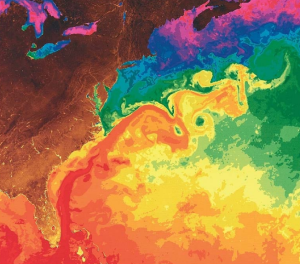
As the Gulf Stream approaches Canada, the current becomes wider and slower as the flow dissipates and it encounters the cold Labrador Current moving in from the north. At this point, the current begins to meander, or change from a fast, straight flow to a slower, looping current (Figure 7.2.2). Often these meanders loop so much that they pinch off and form large rotating water masses called rings or eddies, that separate from the Gulf Stream. If an eddy pinches off from the north side of the Gulf Stream, it entraps a mass of warm water and moves it north into the surrounding cold water of the North Atlantic. These warm core rings are shallow, bowl-shaped water masses about 1 km deep, and about 100 km across, that rotate clockwise as they carry warm water in to the North Atlantic (Figure 7.2.3). If the meanders pinch off at the southern boundary of the Gulf Stream, they form cold core rings that rotate counterclockwise and move to the south. Cold core rings are cone-shaped water masses extending down to over 3.5 km deep, and may be over 500 km wide at the surface.
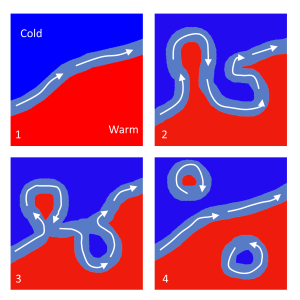
After the Gulf Stream meets the cold Labrador Current, it joins the North Atlantic Current, which transports the warm water towards Europe, where it moderates the European climate. It is estimated that Northern Europe is up to 9o C warmer than expected because of the Gulf Stream, and the warm water helps to keep many northern European ports ice-free in the winter.
In the east, the Gulf Stream merges into the Sargasso Sea, which is the area of the ocean within the rotation center of the North Atlantic gyre. The Sargasso Sea gets its name from the large floating mats of the marine algae Sargassum that are abundant on the surface (Figure 7.2.4). These Sargassum mats may play an important role in the early life stages of sea turtles, who may live and feed within the algae for many years before reaching adulthood.
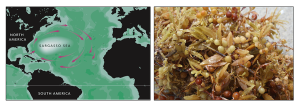
The primary surface current along the east coast of the United States is the Gulf Stream, which was first mapped by Benjamin Franklin in the 18th century (Figure 7.2.1). As a strong, fast current, it reduced the sailing time for ships traveling from the United States back to Europe, so sailors would use thermometers to locate its warm water and stay within the current.
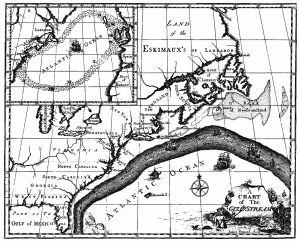
The Gulf Stream is formed from the convergence of the North Atlantic Equatorial Current bringing tropical water from the east, and the Florida Current that brings warm water from the Gulf of Mexico. The Gulf Stream takes this warm water and transports it northwards along the U.S. east coast (Figure 7.2.2). As a western boundary current, the Gulf Stream experiences western intensification (section 7.4), making the current narrow (50-100 km wide), deep (to depths of 1.5 km) and fast. With an average speed of 6.4 km/hr, and a maximum speed of about 9 km/hr, it is the fastest current in the world ocean. It also transports huge amounts of water, more than 100 times greater than the combined flow of all of the rivers on Earth.
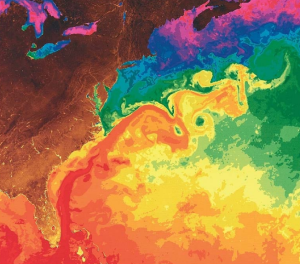
As the Gulf Stream approaches Canada, the current becomes wider and slower as the flow dissipates and it encounters the cold Labrador Current moving in from the north. At this point, the current begins to meander, or change from a fast, straight flow to a slower, looping current (Figure 7.2.2). Often these meanders loop so much that they pinch off and form large rotating water masses called rings or eddies, that separate from the Gulf Stream. If an eddy pinches off from the north side of the Gulf Stream, it entraps a mass of warm water and moves it north into the surrounding cold water of the North Atlantic. These warm core rings are shallow, bowl-shaped water masses about 1 km deep, and about 100 km across, that rotate clockwise as they carry warm water in to the North Atlantic (Figure 7.2.3). If the meanders pinch off at the southern boundary of the Gulf Stream, they form cold core rings that rotate counterclockwise and move to the south. Cold core rings are cone-shaped water masses extending down to over 3.5 km deep, and may be over 500 km wide at the surface.
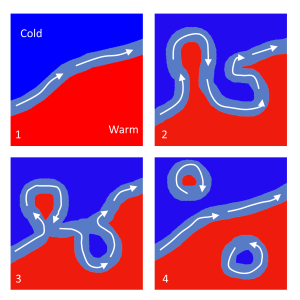
After the Gulf Stream meets the cold Labrador Current, it joins the North Atlantic Current, which transports the warm water towards Europe, where it moderates the European climate. It is estimated that Northern Europe is up to 9o C warmer than expected because of the Gulf Stream, and the warm water helps to keep many northern European ports ice-free in the winter.
In the east, the Gulf Stream merges into the Sargasso Sea, which is the area of the ocean within the rotation center of the North Atlantic gyre. The Sargasso Sea gets its name from the large floating mats of the marine algae Sargassum that are abundant on the surface (Figure 7.2.4). These Sargassum mats may play an important role in the early life stages of sea turtles, who may live and feed within the algae for many years before reaching adulthood.
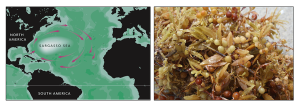
We learned in section 3.3 that refraction causes waves to approach parallel to shore. However, most waves still reach the shore at a small angle, and as each one arrives, it pushes water along the shore, creating what is known as a longshore current within the surf zone (the areas where waves are breaking) (Figure 4.2.1). Longshore currents can move up to 4 km/hr, strong enough to carry people with them, as everyone knows who has been swimming in the ocean only to look up and see that they have been carried far down the beach from their towel!
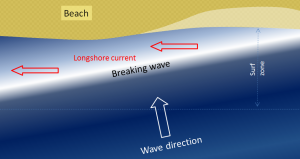
Another important effect of waves reaching the shore at an angle is that when they wash up onto the beach, they do so at an angle, but when that same wave water washes back down the beach, it moves straight down the slope of the beach (Figure 4.2.2). The upward-moving water, known as the swash, pushes sediment particles along the beach, while the downward-moving water, the backwash, brings them straight back. With every wave that washes up and then down the beach, particles of sediment are moved along the beach in a zigzag pattern.
The combined effects of sediment transport within the surf zone by the longshore current and sediment movement along the beach by swash and backwash is known as longshore transport, or littoral drift. Longshore transport moves a tremendous amount of sediment along coasts (both oceans and large lakes) around the world, and it is responsible for creating a variety of depositional features that we will discuss in section 4.4. The net movement of sediment due to longshore transport is to the south along both coasts of the continental United States, because the storms and high winds that originally create the swell tend to occur at higher latitudes and move to the south.
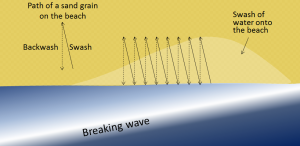
A rip current (often incorrectly called a "rip tide"; they are not really related to tides) is another type of current that develops in the nearshore area, and has the effect of returning water that has been pushed up to the shore by incoming waves or accumulated through longshore currents, particularly converging longshore currents. Rip currents often occur where there is a channel between sandbars that makes it easier for the retreating water to escape. As shown in Figure 4.2.3, rip currents flow straight out from the shore, and because the water is directed through a narrow space, the current can be very strong. The currents lose strength quickly just outside of the surf zone, but they can be dangerous to swimmers who get caught in them and are pulled away from shore. Swimmers caught in a rip current should not try to swim directly back to shore, as it is difficult to fight the current and the swimmer can quickly tire. Instead, swim parallel to the beach for a short distance until you are outside of the rip current, and then you can easily swim to shore.
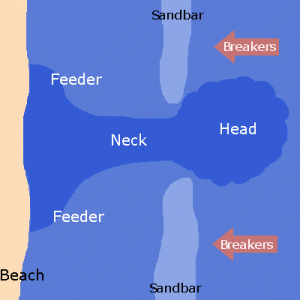
Rip currents are visible in Figure 4.2.4, a beach at Tunquen in Chile near Valparaiso. As is evident from the photo, the rips correspond with embayments in the beach profile. Three of them are indicated with arrows, but it appears that there may be several others farther along the beach.
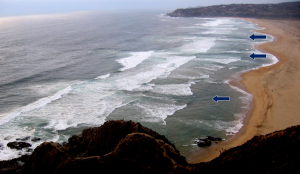
By Paul Webb, used under a CC-BY 4.0 international license. Download this book for free at https://rwu.pressbooks.pub/webboceanography/front-matter/preface/
Modified from "Physical Geology" by Steven Earle used under a CC-BY 4.0 international license. Download this book for free at http://open.bccampus.ca
A whole new ecosystem reliant on the processes of plate tectonics was discovered on the deep seafloor of the Galapagos Rift in 1977. The deep sea submersible Alvin was exploring in 2500 m of water when it encountered unusually warm water. Following the temperature gradient, Alvin eventually discovered jets of superheated water coming from out of the seafloor at temperatures up to 350o C (the normal temperature for water at this depth would be 2-4o C). The water poured out of cracks in the crust, as well as through tall chimneys up to 20 m high and 1 m wide, and as it emerged it took on the appearance of thick black smoke, These fissures were named hydrothermal vents, and the chimneys "black smokers".
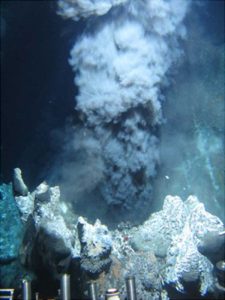
To create these vents, water percolates into the crust where there are plumes of magma close to the surface. The water gets superheated by the magma, then moves back to the surface through convection and is released through the vents. The hot water dissolves minerals from the surrounding rock, and as the water emerges and cools, the dissolved minerals and inorganic sulfides precipitate out as small particles and turn the water black, leading to the black "smoke" coming from the vents. Precipitation of these minerals also create the tall chimneys characteristic of many hydrothermal vents.
Since their original discovery in the Galapagos Rift, hydrothermal vents have been located across the globe along oceanic ridges where there is shallow crust and a lot of tectonic activity (Figure 2.11.2).
Figure 2.11.2 Distribution of hydrothermal vents (red dots) and their association with plate boundaries (By DeDuijn (Own work) [CC BY-SA 4.0], via Wikimedia Commons).
As unexpected as it was to discover these vent systems, even more surprising was the fact that they were teeming with life. The vents are surrounded by a diverse range of previously unknown organisms, including giant tube worms over 2 m long, crabs, shrimp, giant mussels, and mats of bacteria. How is it that such a diverse community can exist in the ocean depths, far removed from the sunlight that supports photosynthesis and primary production in most other ecosystems? The answer is that the water exiting the vents is rich in hydrogen sulfide (H2S), oxygen and CO2. The bacteria surrounding the vents use energy from the oxidation of sulfur compounds like H2S to form carbohydrates from CO2 and water. This is the process of chemosynthesis, and the bacteria are very productive as these reactions occur faster at high temperatures. The bacteria then represent the base of the food web, as other organisms eat the bacteria, or derive their energy from bacteria living symbiotically within their tissues. Watch the video below for more about hydrothermal vents.
https://www.youtube.com/watch?v=UVzBjY8oLkk
Most of the waves discussed in the previous section referred to deep water waves in the open ocean. But what happens when these waves move towards shore and encounter shallow water? Remember that in deep water, a wave’s speed depends on its wavelength, but in shallow water wave speed depends on the depth (section 3.1). When waves approach the shore they will "touch bottom" at a depth equal to half of their wavelength; in other words, when the water depth equals the depth of the wave base (Figure 3.3.1). At this point their behavior will begin to be influenced by the bottom.
When the wave touches the bottom, friction causes the wave to slow down. As one wave slows down, the one behind it catches up to it, thus decreasing the wavelength. However, the wave still contains the same amount of energy, so while the wavelength decreases, the wave height increases. Eventually the wave height exceeds 1/7 of the wavelength, and the wave becomes unstable and forms a breaker. Often breakers will start to curl forwards as they break. This is because the bottom of the wave begins to slow down before the top of the wave, as it is the first part to encounter the seafloor. So the crest of the wave gets “ahead” of the rest of the wave, but has no water underneath it to support it (Figure 3.3.1).
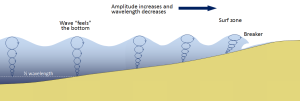
There are three main types of breakers: spilling, plunging, and surging. These are related to the steepness of the bottom, and how quickly the wave will slow down and its energy will get dissipated.
- Spilling breakers form on gently sloping or flatter beaches, where the energy of the wave is dissipated gradually. The wave slowly increases in height, then slowly collapses on itself (Figure 3.3.2). For surfers, these waves provide a longer ride, but they are less exciting.
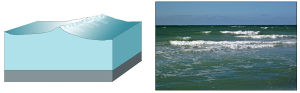
- Plunging breakers form on more steeply-sloped shores, where there is a sudden slowing of the wave and the wave gets higher very quickly. The crest outruns the rest of the wave, curls forwards and breaks with a sudden loss of energy (Figure 3.3.3). These are the “pipeline” waves that surfers seek out.
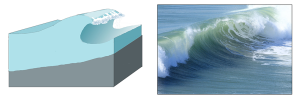
- Surging breakers form on the steepest shorelines. The wave energy is compressed very suddenly right at the shoreline, and the wave breaks right onto the beach (Figure 3.3.4). These waves give too short (and potentially painful) a ride for surfers to enjoy.
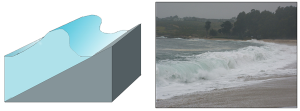
Wave Refraction
Swell can be generated anywhere in the ocean and therefore can arrive at a beach from almost any direction. But if you have ever stood at the shore you have probably noticed that the waves usually approach the shore somewhat parallel to the coast. This is due to wave refraction. If a wave front approaches shore at an angle, the end of the wave front closest to shore will touch bottom before the rest of the wave. This will cause that shallower part of the wave to slow down first, while the rest of the wave that is still in deeper water will continue on at its regular speed. As more and more of the wave front encounters shallower water and slows down, the wave font refracts and the waves tend to align themselves nearly parallel to the shoreline (they are refracted towards the region of slower speed). As we will see in section 5.2, the fact that the waves do not arrive perfectly parallel to the beach causes longshore currents and longshore transport that run parallel to the shore.
Refraction can also explain why waves tend to be larger off of points and headlands, and smaller in bays. A wave front approaching shore will touch the bottom off of the point before it touches bottom in a bay. Once again, the shallower part of the wave front will slow down, and cause the rest of the wave front to refract towards the slower region (the point). Now all of the initial wave energy is concentrated in a relatively small area off of the point, creating large, high energy waves (Figure 3.3.6). In the bay, the refraction has caused the wave fronts to refract away from each other, dispersing the wave energy, and leading to calmer water and smaller waves. This makes the large waves of a “point break” ideal for surfing, while water is calmer in a bay, which is where people would launch a boat. This difference in wave energy also explains why there is net erosion on points, while sand and sediments get deposited in bays (see section 5.3).
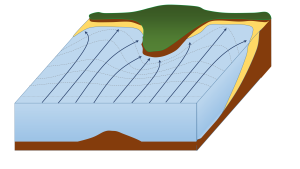
By Paul Webb, used under a CC-BY 4.0 international license. Download this book for free at https://rwu.pressbooks.pub/webboceanography/front-matter/preface/