46 7.1 Surface Gyres
In the previous chapter the major wind patterns on Earth were derived. It is these prevailing winds that blow across the water surface to create the major ocean surface currents. However, only about 2% of the wind energy is actually transferred to the water, so a 50 knot wind only creates a 1 knot current. Furthermore, wind-driven surface currents only affect the top 100-200m of water, meaning surface currents only involve about 10% of the world’s ocean water. In section 7.8 we will examine deep, thermohaline circulation, which impacts around 90% of the ocean water.
Surface currents generally move in the same direction as the winds that created them. However, because of Coriolis deflection, the surface currents are offset approximately 45o relative to the wind direction; 45o to the right in the Northern Hemisphere, and 45o to the left in the Southern Hemisphere. This creates a general circulation pattern where in both hemispheres, surface currents flow east to west between the equator and 30o latitude, west to east between 30o and 60o, and east to west between 60o and the poles (Figure 7.1.1).
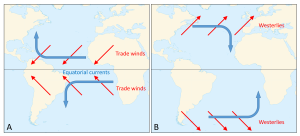
The trade winds create the equatorial currents that flow east to west along the equator; the North Equatorial and South Equatorial currents. If there were no continents, these surface currents would travel all the way around the Earth, parallel to the equator. However, the presence of the continents prevents this unimpeded flow. When these equatorial currents reach the continents, they are diverted and deflected away from the equator by the Coriolis Effect; deflection to the right in the Northern Hemisphere and to the left in the Southern Hemisphere. These currents then become western boundary currents; currents that run along the western side of the ocean basin (i.e. the east coasts of the continents). Since these currents come from the equator, they are warm water currents, bringing warm water to the higher latitudes and distributing heat throughout the ocean.
At the same time, between 30-60o latitude the westerlies move surface water towards the east. The Coriolis Effect and the presence of the continents deflect the currents towards the equator, creating eastern boundary currents (on the eastern side of the ocean basins). These currents come from high latitude areas, so they deliver cold water to the lower latitudes. Together, these currents combine to create large-scale circular patterns of surface circulation called gyres. In the Northern Hemisphere the gyres rotate to the right (clockwise), while in the Southern Hemisphere the gyres rotate to the left (counterclockwise).
There are five major gyres in the oceans; the North Atlantic, South Atlantic, North Pacific, South Pacific, and Indian (Figure 7.1.2). The North Pacific gyre is composed of the North Equatorial Current on its southern boundary, which turns into the Kuroshio Current (a.k.a. the Japan Current) bringing warm water north towards Japan. The Kuroshio flows into the North Pacific Current which moves east towards North America, where it becomes the California Current to complete the gyre. The North Atlantic gyre is formed by the North Equatorial Current flowing into the Gulf Stream along the east coast of the United States. The Gulf Stream merges into the North Atlantic Current to move water towards Europe, which then becomes the Canary Current as it moves south to join the North Equatorial Current.
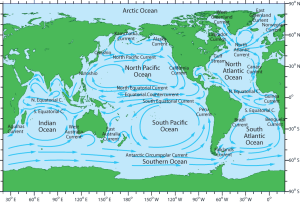
Near Antarctica the circulation is somewhat different. Because there is little in the way of continental land masses between 50-60o south, the surface current created by the westerly winds can make its way completely around the Earth, creating the Antarctic Circumpolar Current (ACC) or West Wind Drift (WWD) that flows from west to east (Figure 7.1.2). The Antarctic Circumpolar Current is the only current that connects all of the major ocean basins, and in terms of the amount of water that it transports, it is the largest surface current on Earth. Above 60o latitude the prevailing winds are the polar easterlies, which create a current flowing from east to west along the edge of the Antarctic continent, the East Wind Drift or the Antarctic Coastal Current.
The Antarctic Circumpolar Current creates the southern boundary for all of the Southern Hemisphere gyres. In the South Pacific gyre the ACC becomes the Peru Current (also known as the Humboldt Current) moving up the west coast of South America, before joining the South Equatorial Current. The South Equatorial Current flows southwards as the East Australia Current, before completing the gyre with the ACC. The South Atlantic gyre is composed of the South Equatorial Current, the Brazil Current, the ACC, and the Benguela Current. Finally, the currents making up the Indian gyre are the ACC, the West Australia Current, the South Equatorial Current, and the Agulhas Current.
Not all of the equatorial water that is moved westward by the trade winds and reaches the continents gets transported to higher latitudes in the gyres, because the Coriolis Effect is weakest along the equator. Instead, some of the water piles up along the western edge of the ocean, and then flows eastward due to gravity, creating narrow Equatorial Countercurrents between the North and South Equatorial Currents (Figure 7.1.2). Some of this water also moves east as equatorial undercurrents that flow at depths between 50-200 m, underneath the Equatorial Currents. These undercurrents are called the Lomonosov Current in the Atlantic, and the Cromwell Current in the Pacific.
Convergent boundaries, where two plates are moving toward each other, are of three types, depending on the type of crust present on either side of the boundary — oceanic or continental. The types are ocean-ocean, ocean-continent, and continent-continent.
At an ocean-ocean convergent boundary, one of the plates (oceanic crust and lithospheric mantle) is pushed, or subducted, under the other (Figure 2.6.1). Often it is the older and colder plate that is denser and subducts beneath the younger and warmer plate. There is commonly an ocean trench along the boundary as the crust bends downwards. The subducted lithosphere descends into the hot mantle at a relatively shallow angle close to the subduction zone, but at steeper angles farther down (up to about 45°). The significant volume of water within the subducting material is released as the subducting crust is heated. It mixes with the overlying mantle, and the addition of water to the hot mantle lowers the crust’s melting point and leads to the formation of magma (flux melting). The magma, which is lighter than the surrounding mantle material, rises through the mantle and the overlying oceanic crust to the ocean floor where it creates a chain of volcanic islands known as an island arc. A mature island arc develops into a chain of relatively large islands (such as Japan or Indonesia) as more and more volcanic material is extruded and sedimentary rocks accumulate around the islands. Earthquakes occur relatively deep below the seafloor, where the subducting crust moves against the overriding crust.
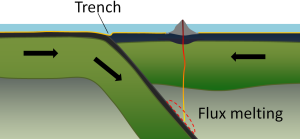
Examples of ocean-ocean convergent zones are subduction of the Pacific Plate south of Alaska (creating the Aleutian Islands) and under the Philippine Plate, where it creates the Marianas Trench, the deepest part of the ocean.
At an ocean-continent convergent boundary, the denser oceanic plate is pushed under the less dense continental plate in the same manner as at an ocean-ocean boundary. Sediment that has accumulated on the seafloor is thrust up into an accretionary wedge, and compression leads to thrusting within the continental plate (Figure 2.6.2). The magma produced adjacent to the subduction zone rises to the base of the continental crust and leads to partial melting of the crustal rock. The resulting magma ascends through the crust, producing a mountain chain with many volcanoes. As with an ocean-ocean boundary, the subducting crust can produce a deep trench running parallel to the coastline.
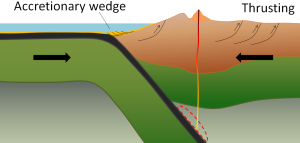
Examples of ocean-continent convergent boundaries are subduction of the Nazca Plate under South America (which has created the Andes Mountains and the Peru Trench) and subduction of the Juan de Fuca Plate under North America (creating the Cascade Range).
A continent-continent collision occurs when a continent or large island that has been moved along with subducting oceanic crust collides with another continent (Figure 2.6.3). The colliding continental material will not be subducted because it is too light (i.e., because it is composed largely of light continental rocks), but the root of the oceanic plate will eventually break off and sink into the mantle. There is tremendous deformation of the pre-existing continental rocks, forcing the material upwards and creating mountains.

Examples of continent-continent convergent boundaries are the collision of the India Plate with the Eurasian Plate, creating the Himalaya Mountains, and the collision of the African Plate with the Eurasian Plate, creating the series of ranges extending from the Alps in Europe to the Zagros Mountains in Iran.
With so many variables playing a role in the production of tides, it is understandable that not every place on Earth will experience exactly the same tidal conditions. There are three primary classifications for tides, depending on the number and relative heights of tidal cycles per day.
A diurnal tide consists of only one high tide and one low tide per day (Figure 3.7.1). "Diurnal" refers to a daily occurrence, so a situation where there is only one complete tidal cycle per day is considered a diurnal tide. Diurnal tides are common in the Gulf of Mexico, along the west coast of Alaska, and in parts of Southeast Asia.
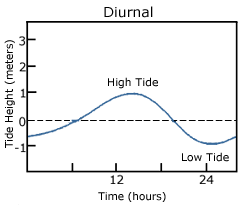
A semidiurnal tide exhibits two high and two low tides each day, with both highs and both lows of toughly equal height (Figure 3.7.2). "Semidiurnal" means "half of a day"; one tidal cycle takes half of a day, therefore there are two complete cycles per day. Semidiurnal tides are common along the east coasts of North America and Australia, the west coast of Africa, and most of Europe.
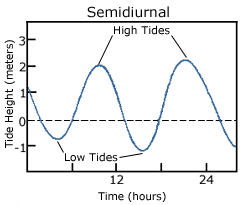
Mixed semidiurnal tides (or mixed tides), have two high tides and two low tides per day, but the heights of each tide differs; the two high tides are of different heights, as are the two low tides (Figure 3.7.3). The differences in height may be the result of amphidromic circulation, the angle of the moon, or any of the other variables discussed in section 3.6. Mixed semidiurnal tides are found along the Pacific coast of North America.
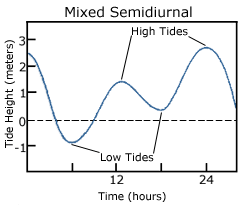
Figure 3.7.4 shows the distribution of the various tide types throughout the world.

Tidal Currents
The movement of water with the rising and falling tide creates tidal currents. As the tide rises, water flows into an area, creating a flood current. As the tide falls and water flows out an ebb current is created. Slack water, or slack tides occur during the transition between incoming high and outgoing low tides, when there is no net water movement.
The strength of a tidal current depends on the volume of water that enters and exits with each tidal cycle (the tidal volume or tidal prism), and the area through which the water flows. A large tidal volume moving through a large area may create only a weak tidal current, as the volume is spread over a wide area. On the other hand, a narrow area may produce a strong tidal current even if the tidal volume is small, as all of the water is forced through a small area. It follows that the strongest tidal currents will result from a large tidal range moving through a narrow area.
Tidal bores occur where rivers meet the ocean. If the incoming tidal current is stronger than the river outflow, the tidal bore appears as a wave, or moving wall of water that moves up the river as the tide comes in (Figure 3.7.5).

In many cases these tidal bores may move through a river or inlet for many kilometers, and if they are large enough they can form continually breaking waves that surfers can ride much farther and longer than a traditional ocean wave, such as the Severn Bore in England, shown in the video below.
https://youtu.be/IKA39LQOIck
Additional links for more information
- For an even more dramatic tidal bore, watch this video of the "Silver Dragon" on China's Qiantang River
By Paul Webb, used under a CC-BY 4.0 international license. Download this book for free at https://rwu.pressbooks.pub/webboceanography/front-matter/preface/
In the previous section we learned that rising air creates low pressure systems, and sinking air creates high pressure. In addition to their role in creating the surface winds, these high and low pressure systems also influence other climatic phenomena. Along the equator air is rising as it is warmed by solar radiation (section 6.2). Warm air contains more water vapor than cold air, which is why we experience humidity during the summer and not during the winter. The water content of air roughly doubles with every 10o C increase in temperature. So the air rising at the equator is warm and full of water vapor; as it rises into the upper atmosphere it cools, and the cool air can no longer hold as much water vapor, so the water condenses and forms rain. Therefore, low pressure systems are associated with precipitation, and we see wet habitats like tropical rainforests near the equator (Figure 6.3.1).
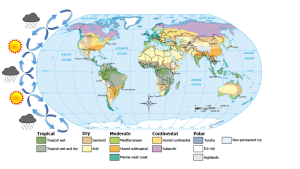
After rising and producing rain near the equator, the air masses move towards 30o latitude and sink back towards Earth as part of the Hadley convection cells. This air has lost most of its moisture after producing the equatorial rains, so the sinking air is dry, resulting in arid climates near 30o latitude in both hemispheres. Many of the major desert regions on Earth are located near 30o latitude, including much of Australia, the Middle East, and the Sahara Desert of Africa (Figure 6.3.1). The air also becomes compressed and heats up as it sinks, absorbing any moisture from the clouds and creating clear skies. Thus high pressure systems are associated with dry weather and clear skies. This cycle of high and low pressure regions continues with the Ferrel and Polar convection cells, leading to rain and the boreal forests at 60o latitude in the Northern Hemisphere (there are no corresponding large land masses at these latitudes in the Southern Hemisphere). At the poles, descending, dry air produces little precipitation, leading to the polar desert climate.
The elevation of the land also plays a role in precipitation and climactic characteristics. As moist air moves over land and encounters mountains it rises, expands, and cools because of the declining pressure and temperature. The cool air holds less water vapor, so condensation occurs and rain falls on the windward side of the mountains. As the air passes over the mountains to the leeward side, it is now dry air, and as it sinks the pressure increases, it heats back up, any moisture revaporizes, and it creates dry, deserts regions behind the mountains (Figure 6.3.2). This phenomenon is referred to as a rain shadow, and can be found in areas such as the Tibetan Plateau and Gobi Desert behind the Himalayas, Death Valley behind the Sierra Nevada mountains, and the dry San Joaquin Valley in California.
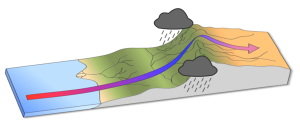
Rising and falling air are also responsible for more localized, short-term wind patterns in coastal areas. Due to the high heat capacity of water, land heats up and cools down about five times faster than water. During the day the sun heats up the land faster than it heats the water, setting up a convection cell of warmer rising air over the land and sinking cooler air over the water. This creates winds blowing from the water towards the land during the day and early evening; a sea breeze (Figure 6.3.3). The opposite occurs at night, when the land cools more quickly than the ocean. Now the ocean is warmer than the land, so air rises over the water and sinks over the land, creating a convection cell where winds blow from land towards the water. This is a land breeze, which blows at night and into the early morning (Figure 6.3.3).
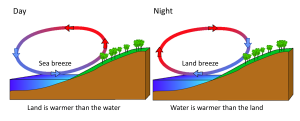
The same phenomenon leads to seasonal climatic changes in many areas. During the winter the lower pressure is over the warmer ocean, and the high pressure is over the colder land, so winds blow from land to sea. In summer the land is warmer than the ocean, causing low pressure over the land and winds to blow from the ocean towards the land. The winds blowing from the ocean contain a lot of water vapor, and as the moist air passes over land and rises, it cools and condenses causing seasonal rains, such as the summer monsoons of southeast Asia (Figure 6.3.4).

The primary surface current along the east coast of the United States is the Gulf Stream, which was first mapped by Benjamin Franklin in the 18th century (Figure 7.2.1). As a strong, fast current, it reduced the sailing time for ships traveling from the United States back to Europe, so sailors would use thermometers to locate its warm water and stay within the current.
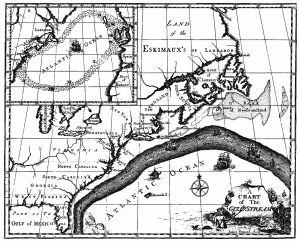
The Gulf Stream is formed from the convergence of the North Atlantic Equatorial Current bringing tropical water from the east, and the Florida Current that brings warm water from the Gulf of Mexico. The Gulf Stream takes this warm water and transports it northwards along the U.S. east coast (Figure 7.2.2). As a western boundary current, the Gulf Stream experiences western intensification (section 7.4), making the current narrow (50-100 km wide), deep (to depths of 1.5 km) and fast. With an average speed of 6.4 km/hr, and a maximum speed of about 9 km/hr, it is the fastest current in the world ocean. It also transports huge amounts of water, more than 100 times greater than the combined flow of all of the rivers on Earth.
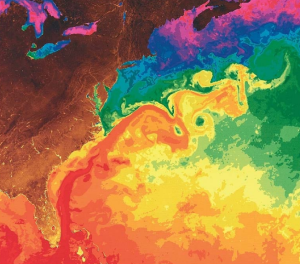
As the Gulf Stream approaches Canada, the current becomes wider and slower as the flow dissipates and it encounters the cold Labrador Current moving in from the north. At this point, the current begins to meander, or change from a fast, straight flow to a slower, looping current (Figure 7.2.2). Often these meanders loop so much that they pinch off and form large rotating water masses called rings or eddies, that separate from the Gulf Stream. If an eddy pinches off from the north side of the Gulf Stream, it entraps a mass of warm water and moves it north into the surrounding cold water of the North Atlantic. These warm core rings are shallow, bowl-shaped water masses about 1 km deep, and about 100 km across, that rotate clockwise as they carry warm water in to the North Atlantic (Figure 7.2.3). If the meanders pinch off at the southern boundary of the Gulf Stream, they form cold core rings that rotate counterclockwise and move to the south. Cold core rings are cone-shaped water masses extending down to over 3.5 km deep, and may be over 500 km wide at the surface.
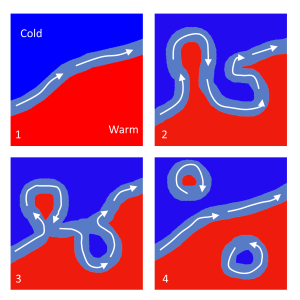
After the Gulf Stream meets the cold Labrador Current, it joins the North Atlantic Current, which transports the warm water towards Europe, where it moderates the European climate. It is estimated that Northern Europe is up to 9o C warmer than expected because of the Gulf Stream, and the warm water helps to keep many northern European ports ice-free in the winter.
In the east, the Gulf Stream merges into the Sargasso Sea, which is the area of the ocean within the rotation center of the North Atlantic gyre. The Sargasso Sea gets its name from the large floating mats of the marine algae Sargassum that are abundant on the surface (Figure 7.2.4). These Sargassum mats may play an important role in the early life stages of sea turtles, who may live and feed within the algae for many years before reaching adulthood.
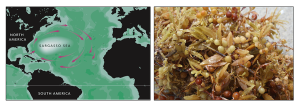
Learning Objectives
After reading this chapter you should be able to:
- identify the major ocean surface currents of the world (i.e. the gyres) and explain how they are formed
- identify the features of the Gulf Stream, including the formation of warm and cold core rings
- explain the development and consequences of the Ekman spiral
- explain geostrophic flow, and how it helps keep the gyres flowing even when wind dies down.
- explain why gyre currents are more intense on the western side of the oceans (western intensification)
- explain the causes behind upwelling and downwelling, and the impacts of these events on primary production
- identify the locations of some of the major upwelling regions on Earth
- explain the causes and effects of ENSO events
- explain Langmuir cells
- explain the processes that drive thermohaline circulation
- interpret a T-S diagram to identify water masses
- identify the major global sites of deep water formation
- identify the major global water masses
- explain how deep water circulates throughout the world ocean
Ocean waters are constantly in motion, from ocean-scale surface currents, to density-driven vertical turnover, to small rotating eddies. Oceanographers have an array of sophisticated tools to measure ocean currents, but from time to time, fortuitous accidents can also aid our understanding of ocean circulation. A great example is the case of the container ship Ever Laurel, which was on its way from Hong Kong to Tacoma, Washington, in January 1992, when 12 containers were washed overboard in a storm in the middle of the Pacific. One of the containers contained over 28,000 plastic bath toys, which were released into the ocean as the container hit the water. Ten months later, the bath toys began washing ashore, first near Sitka, Alaska, then elsewhere along the Alaskan coast, and by 1996, in Washington. Over the next two decades, some toys traveled as far as the Pacific coasts of South America and Australia, while others were found in the Arctic ice, and some even made it through the Arctic into the North Atlantic, washing up in Newfoundland and Scotland (Figure 10.1). There are still a few thousand of the toys floating around in the central North Pacific, and the paths taken by all of these toys have allowed oceanographers to study the movements of large-scale ocean surface currents.
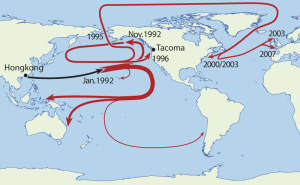
By Paul Webb, used under a CC-BY 4.0 international license. Download this book for free at https://rwu.pressbooks.pub/webboceanography/front-matter/preface/
Most of the waves discussed in the previous section referred to deep water waves in the open ocean. But what happens when these waves move towards shore and encounter shallow water? Remember that in deep water, a wave’s speed depends on its wavelength, but in shallow water wave speed depends on the depth (section 3.1). When waves approach the shore they will "touch bottom" at a depth equal to half of their wavelength; in other words, when the water depth equals the depth of the wave base (Figure 3.3.1). At this point their behavior will begin to be influenced by the bottom.
When the wave touches the bottom, friction causes the wave to slow down. As one wave slows down, the one behind it catches up to it, thus decreasing the wavelength. However, the wave still contains the same amount of energy, so while the wavelength decreases, the wave height increases. Eventually the wave height exceeds 1/7 of the wavelength, and the wave becomes unstable and forms a breaker. Often breakers will start to curl forwards as they break. This is because the bottom of the wave begins to slow down before the top of the wave, as it is the first part to encounter the seafloor. So the crest of the wave gets “ahead” of the rest of the wave, but has no water underneath it to support it (Figure 3.3.1).
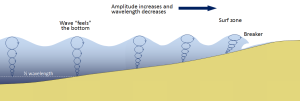
There are three main types of breakers: spilling, plunging, and surging. These are related to the steepness of the bottom, and how quickly the wave will slow down and its energy will get dissipated.
- Spilling breakers form on gently sloping or flatter beaches, where the energy of the wave is dissipated gradually. The wave slowly increases in height, then slowly collapses on itself (Figure 3.3.2). For surfers, these waves provide a longer ride, but they are less exciting.
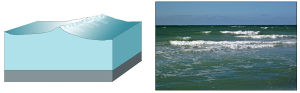
- Plunging breakers form on more steeply-sloped shores, where there is a sudden slowing of the wave and the wave gets higher very quickly. The crest outruns the rest of the wave, curls forwards and breaks with a sudden loss of energy (Figure 3.3.3). These are the “pipeline” waves that surfers seek out.
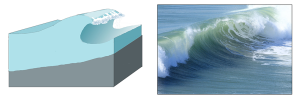
- Surging breakers form on the steepest shorelines. The wave energy is compressed very suddenly right at the shoreline, and the wave breaks right onto the beach (Figure 3.3.4). These waves give too short (and potentially painful) a ride for surfers to enjoy.
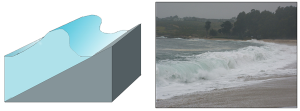
Wave Refraction
Swell can be generated anywhere in the ocean and therefore can arrive at a beach from almost any direction. But if you have ever stood at the shore you have probably noticed that the waves usually approach the shore somewhat parallel to the coast. This is due to wave refraction. If a wave front approaches shore at an angle, the end of the wave front closest to shore will touch bottom before the rest of the wave. This will cause that shallower part of the wave to slow down first, while the rest of the wave that is still in deeper water will continue on at its regular speed. As more and more of the wave front encounters shallower water and slows down, the wave font refracts and the waves tend to align themselves nearly parallel to the shoreline (they are refracted towards the region of slower speed). As we will see in section 5.2, the fact that the waves do not arrive perfectly parallel to the beach causes longshore currents and longshore transport that run parallel to the shore.
Refraction can also explain why waves tend to be larger off of points and headlands, and smaller in bays. A wave front approaching shore will touch the bottom off of the point before it touches bottom in a bay. Once again, the shallower part of the wave front will slow down, and cause the rest of the wave front to refract towards the slower region (the point). Now all of the initial wave energy is concentrated in a relatively small area off of the point, creating large, high energy waves (Figure 3.3.6). In the bay, the refraction has caused the wave fronts to refract away from each other, dispersing the wave energy, and leading to calmer water and smaller waves. This makes the large waves of a “point break” ideal for surfing, while water is calmer in a bay, which is where people would launch a boat. This difference in wave energy also explains why there is net erosion on points, while sand and sediments get deposited in bays (see section 5.3).
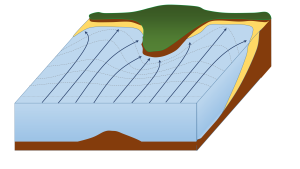
By Paul Webb, used under a CC-BY 4.0 international license. Download this book for free at https://rwu.pressbooks.pub/webboceanography/front-matter/preface/
Learning Objectives
After reading this chapter you should be able to:
- identify the major ocean surface currents of the world (i.e. the gyres) and explain how they are formed
- identify the features of the Gulf Stream, including the formation of warm and cold core rings
- explain the development and consequences of the Ekman spiral
- explain geostrophic flow, and how it helps keep the gyres flowing even when wind dies down.
- explain why gyre currents are more intense on the western side of the oceans (western intensification)
- explain the causes behind upwelling and downwelling, and the impacts of these events on primary production
- identify the locations of some of the major upwelling regions on Earth
- explain the causes and effects of ENSO events
- explain Langmuir cells
- explain the processes that drive thermohaline circulation
- interpret a T-S diagram to identify water masses
- identify the major global sites of deep water formation
- identify the major global water masses
- explain how deep water circulates throughout the world ocean
Ocean waters are constantly in motion, from ocean-scale surface currents, to density-driven vertical turnover, to small rotating eddies. Oceanographers have an array of sophisticated tools to measure ocean currents, but from time to time, fortuitous accidents can also aid our understanding of ocean circulation. A great example is the case of the container ship Ever Laurel, which was on its way from Hong Kong to Tacoma, Washington, in January 1992, when 12 containers were washed overboard in a storm in the middle of the Pacific. One of the containers contained over 28,000 plastic bath toys, which were released into the ocean as the container hit the water. Ten months later, the bath toys began washing ashore, first near Sitka, Alaska, then elsewhere along the Alaskan coast, and by 1996, in Washington. Over the next two decades, some toys traveled as far as the Pacific coasts of South America and Australia, while others were found in the Arctic ice, and some even made it through the Arctic into the North Atlantic, washing up in Newfoundland and Scotland (Figure 10.1). There are still a few thousand of the toys floating around in the central North Pacific, and the paths taken by all of these toys have allowed oceanographers to study the movements of large-scale ocean surface currents.
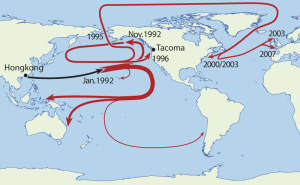
By Paul Webb, used under a CC-BY 4.0 international license. Download this book for free at https://rwu.pressbooks.pub/webboceanography/front-matter/preface/