44 6.4 Hurricanes
The most dramatic examples of low pressure systems leading to storms and rain are hurricanes, cyclones, and typhoons. All three of these terms describe the same atmospheric processes and the same types of storms; it’s just that different terminology is used in different parts of the world. In the Atlantic and Northeast Pacific, the storms are called hurricanes, in the Indian and South Pacific Oceans they are referred to as cyclones, and they are called typhoons in the Northwest Pacific.
Hurricanes begin as low pressure systems formed over warm, tropical water. They only form in tropical regions because they need the heat from the warm water to fuel the storm. The warm, moist air rises, cools, and condenses, forming rain, and the condensation releases more latent heat into the atmosphere. This heat causes even more air to rise and condense, further fueling the storm.
As the air rises towards the center of the storm, more warm tropical air rushes in to replace it, causing very strong winds. But the air does not move directly towards the center of the storm. Because of the large size of hurricanes, the air rushing towards the center will be deflected by the Coriolis Effect, causing the entire storm to rotate. In the Northern Hemisphere that deflection is to the right, causing Northern Hemisphere hurricanes to rotate counterclockwise. In the Southern Hemisphere, the winds are deflected to the left, leading to a clockwise rotation (Figure 6.4.1).
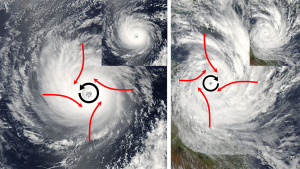
The violent winds characteristic of hurricanes are the result of the spiraling air that is moving towards the center of the storm, and once its winds exceed 74 mph the storm officially becomes a hurricane. At the very center of the hurricane, the pressure is so low that cool, dry air from the upper atmosphere get sucked downwards, leading to a central region of calm, clear skies; the hurricane’s eye (Figure 6.4.2).
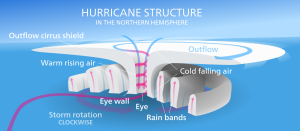
Hurricanes in the North Atlantic form as tropical storms over the warm water off of the African coast, and are moved east to west by the trade winds (Figure 6.4.3). As the storms move west over the tropical ocean, their energy increases until they reach hurricane status. As they approach the Caribbean, the Coriolis Effect deflects their path to the right, causing them to move towards the north (Figure 6.4.3). Eventually hurricanes might make landfall, causing extensive damage to coastal areas through the high winds, rain, and flooding. However, hurricanes often die out fairly soon after reaching land. When a storm moves over land it becomes cut off from the warm moist ocean air that has sustained it. Without that fuel source, the storm loses power and begins to dissipate.
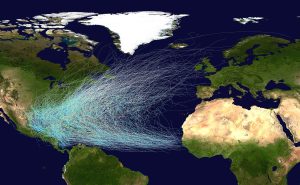
A similar pattern occurs in the Pacific and in the Southern Hemisphere. The trade winds move the storms from east to west, and they are deflected as they approach the coasts; to the right in the Northern Hemisphere and to the left in the Southern Hemisphere (Figure 6.4.4).
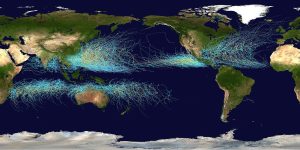
While the very high winds and intense rain of hurricanes can cause significant damage, in many cases it is the storm surge that leads to the most death and destruction. The storm surge is a “hill” of water that forms on the ocean surface below a hurricane. The surge is the result of two processes; a small hill is produced due to the extreme low pressure in the eye of a hurricane, which pulls water upwards towards the eye, creating a pressure surge. A larger surge is produced by the winds blowing and piling up water in the direction the storm is traveling (Figure 6.4.5). As the hurricane makes landfall, the effect of the storm surge is equivalent to a very large and sudden rise in sea level as the surge moves over the land, causing extensive flooding.
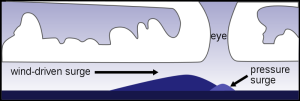
In 1970 the Bhola Cyclone struck Bangladesh with a 40 ft. storm surge, leading to the death of about 500,000 people, the deadliest hurricane in history. The east coast of the United States was hit by the New England Hurricane of 1938, which had a 16 ft. storm surge and left almost 700 people dead.
Preventing Storm Surge Damage
In response to the hurricane-related tragedies like those listed above, may cities have built hurricane barriers designed to reduce the flooding and damage associated with storm surges. Downtown Providence, Rhode Island, USA, was submerged under 13 feet of water during the Great New England hurricane of 1938, and was flooded again following Hurricane Carol in 1954. In the 1960s the Fox Point Hurricane Barrier was constructed at the mouth of the Providence River. It consists of a high wall with three “doors” that are left open under normal conditions, but can be closed during a hurricane to prevent a storm surge of up to 20.5 feet from inundating the city (Figure 6.4.6, left). A related concept is seen in the storm surge barrier on the Hollandse IJssel river in the Netherlands, where the barrier is lowered to prevent flooding (Figure 6.4.6, right).
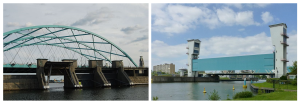
Convergent boundaries, where two plates are moving toward each other, are of three types, depending on the type of crust present on either side of the boundary — oceanic or continental. The types are ocean-ocean, ocean-continent, and continent-continent.
At an ocean-ocean convergent boundary, one of the plates (oceanic crust and lithospheric mantle) is pushed, or subducted, under the other (Figure 2.6.1). Often it is the older and colder plate that is denser and subducts beneath the younger and warmer plate. There is commonly an ocean trench along the boundary as the crust bends downwards. The subducted lithosphere descends into the hot mantle at a relatively shallow angle close to the subduction zone, but at steeper angles farther down (up to about 45°). The significant volume of water within the subducting material is released as the subducting crust is heated. It mixes with the overlying mantle, and the addition of water to the hot mantle lowers the crust’s melting point and leads to the formation of magma (flux melting). The magma, which is lighter than the surrounding mantle material, rises through the mantle and the overlying oceanic crust to the ocean floor where it creates a chain of volcanic islands known as an island arc. A mature island arc develops into a chain of relatively large islands (such as Japan or Indonesia) as more and more volcanic material is extruded and sedimentary rocks accumulate around the islands. Earthquakes occur relatively deep below the seafloor, where the subducting crust moves against the overriding crust.
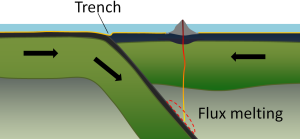
Examples of ocean-ocean convergent zones are subduction of the Pacific Plate south of Alaska (creating the Aleutian Islands) and under the Philippine Plate, where it creates the Marianas Trench, the deepest part of the ocean.
At an ocean-continent convergent boundary, the denser oceanic plate is pushed under the less dense continental plate in the same manner as at an ocean-ocean boundary. Sediment that has accumulated on the seafloor is thrust up into an accretionary wedge, and compression leads to thrusting within the continental plate (Figure 2.6.2). The magma produced adjacent to the subduction zone rises to the base of the continental crust and leads to partial melting of the crustal rock. The resulting magma ascends through the crust, producing a mountain chain with many volcanoes. As with an ocean-ocean boundary, the subducting crust can produce a deep trench running parallel to the coastline.
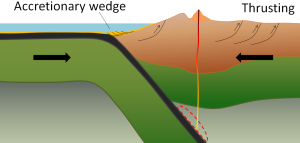
Examples of ocean-continent convergent boundaries are subduction of the Nazca Plate under South America (which has created the Andes Mountains and the Peru Trench) and subduction of the Juan de Fuca Plate under North America (creating the Cascade Range).
A continent-continent collision occurs when a continent or large island that has been moved along with subducting oceanic crust collides with another continent (Figure 2.6.3). The colliding continental material will not be subducted because it is too light (i.e., because it is composed largely of light continental rocks), but the root of the oceanic plate will eventually break off and sink into the mantle. There is tremendous deformation of the pre-existing continental rocks, forcing the material upwards and creating mountains.

Examples of continent-continent convergent boundaries are the collision of the India Plate with the Eurasian Plate, creating the Himalaya Mountains, and the collision of the African Plate with the Eurasian Plate, creating the series of ranges extending from the Alps in Europe to the Zagros Mountains in Iran.
In the previous section we learned that rising air creates low pressure systems, and sinking air creates high pressure. In addition to their role in creating the surface winds, these high and low pressure systems also influence other climatic phenomena. Along the equator air is rising as it is warmed by solar radiation (section 6.2). Warm air contains more water vapor than cold air, which is why we experience humidity during the summer and not during the winter. The water content of air roughly doubles with every 10o C increase in temperature. So the air rising at the equator is warm and full of water vapor; as it rises into the upper atmosphere it cools, and the cool air can no longer hold as much water vapor, so the water condenses and forms rain. Therefore, low pressure systems are associated with precipitation, and we see wet habitats like tropical rainforests near the equator (Figure 6.3.1).
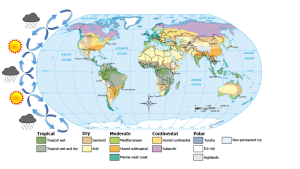
After rising and producing rain near the equator, the air masses move towards 30o latitude and sink back towards Earth as part of the Hadley convection cells. This air has lost most of its moisture after producing the equatorial rains, so the sinking air is dry, resulting in arid climates near 30o latitude in both hemispheres. Many of the major desert regions on Earth are located near 30o latitude, including much of Australia, the Middle East, and the Sahara Desert of Africa (Figure 6.3.1). The air also becomes compressed and heats up as it sinks, absorbing any moisture from the clouds and creating clear skies. Thus high pressure systems are associated with dry weather and clear skies. This cycle of high and low pressure regions continues with the Ferrel and Polar convection cells, leading to rain and the boreal forests at 60o latitude in the Northern Hemisphere (there are no corresponding large land masses at these latitudes in the Southern Hemisphere). At the poles, descending, dry air produces little precipitation, leading to the polar desert climate.
The elevation of the land also plays a role in precipitation and climactic characteristics. As moist air moves over land and encounters mountains it rises, expands, and cools because of the declining pressure and temperature. The cool air holds less water vapor, so condensation occurs and rain falls on the windward side of the mountains. As the air passes over the mountains to the leeward side, it is now dry air, and as it sinks the pressure increases, it heats back up, any moisture revaporizes, and it creates dry, deserts regions behind the mountains (Figure 6.3.2). This phenomenon is referred to as a rain shadow, and can be found in areas such as the Tibetan Plateau and Gobi Desert behind the Himalayas, Death Valley behind the Sierra Nevada mountains, and the dry San Joaquin Valley in California.
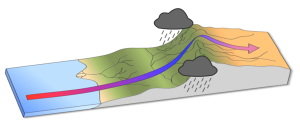
Rising and falling air are also responsible for more localized, short-term wind patterns in coastal areas. Due to the high heat capacity of water, land heats up and cools down about five times faster than water. During the day the sun heats up the land faster than it heats the water, setting up a convection cell of warmer rising air over the land and sinking cooler air over the water. This creates winds blowing from the water towards the land during the day and early evening; a sea breeze (Figure 6.3.3). The opposite occurs at night, when the land cools more quickly than the ocean. Now the ocean is warmer than the land, so air rises over the water and sinks over the land, creating a convection cell where winds blow from land towards the water. This is a land breeze, which blows at night and into the early morning (Figure 6.3.3).
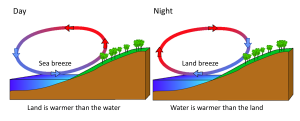
The same phenomenon leads to seasonal climatic changes in many areas. During the winter the lower pressure is over the warmer ocean, and the high pressure is over the colder land, so winds blow from land to sea. In summer the land is warmer than the ocean, causing low pressure over the land and winds to blow from the ocean towards the land. The winds blowing from the ocean contain a lot of water vapor, and as the moist air passes over land and rises, it cools and condenses causing seasonal rains, such as the summer monsoons of southeast Asia (Figure 6.3.4).

Radiant energy from the sun is important for several major oceanic processes:
- Climate, winds, and major ocean currents are ultimately dependent on solar radiation reaching the Earth and heating different areas to different degrees.
- Sunlight warms the surface water where much oceanic life lives.
- Solar radiation provides light for photosynthesis, which supports the entire ocean ecosystem.
The energy reaching Earth from the sun is a form of electromagnetic radiation, which is represented by the electromagnetic spectrum (Figure 5.9.1). Electromagnetic waves vary in their frequency and wavelength. High frequency waves have very short wavelengths, and are very high energy forms of radiation, such as gamma rays and x-rays. These rays can easily penetrate the bodies of living organisms and interfere with individual atoms and molecules. At the other end of the spectrum are low energy, long wavelength waves such as radio waves, which do not pose a hazard to living organisms.
Most of the solar energy reaching the Earth is in the range of visible light, with wavelengths between about 400-700 nm. Each color of visible light has a unique wavelength, and together they make up white light. The shortest wavelengths are on the violet and ultraviolet end of the spectrum, while the longest wavelengths are at the red and infrared end. In between, the colors of the visible spectrum comprise the familiar "ROYGBIV"; red, orange, yellow, green, blue, indigo, and violet.
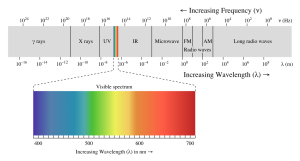
Water is very effective at absorbing incoming light, so the amount of light penetrating the ocean declines rapidly (is attenuated) with depth (Figure 5.9.2). At 1 m depth, only 45% of the solar energy that falls on the ocean surface remains. At 10 m depth only 16% of the light is still present, and only 1% of the original light is left at 100 m. No light penetrates beyond 1000 m.
In addition to overall attenuation, the oceans absorb the different wavelengths of light at different rates (Figure 5.9.2). The wavelengths at the extreme ends of the visible spectrum are attenuated faster than those wavelengths in the middle. Longer wavelengths are absorbed first; red is absorbed in the upper 10 m, orange by about 40 m, and yellow disappears before 100 m. Shorter wavelengths penetrate further, with blue and green light reaching the deepest depths.
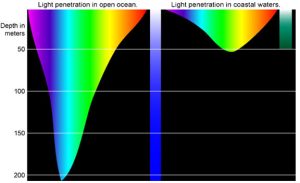
This explains why everything appears blue under water. The colors we perceive depends on the wavelengths of light that are received by our eyes. If an object appears red to us, that is because the object reflects red light but absorbs all of the other colors. So the only color reaching our eyes is red. Under water, blue is the only color of light still available at depth, so that is the only color that can be reflected back to our eyes, and everything has a blue tinge under water. A red object at depth will not appear red to us because there is no red light available to reflect off of the object. Objects in water will only appear as their real colors near the surface where all wavelengths of light are still available, or if the other wavelengths of light are provided artificially, such as by illuminating the object with a dive light.
Water in the open ocean appears clear and blue because it contains much less particulate matter, such as phytoplankton or other suspended particles, and the clearer the water, the deeper the light penetration. Blue light penetrates deeply and is scattered by the water molecules, while all other colors are absorbed; thus the water appears blue. On the other hand, coastal water often appears greenish (Figure 5.9.2). Coastal water contains much more suspended silt and algae and microscopic organisms than the open ocean. Many of these organisms, such as phytoplankton, absorb light in the blue and red range through their photosynthetic pigments, leaving green as the dominant wavelength of reflected light. Therefore the higher the phytoplankton concentration in water, the greener it appears. Small silt particles may also absorb blue light, further shifting the color of water away from blue when there are high concentrations of suspended particles.
The ocean can be divided into depth layers depending on the amount of light penetration, as discussed in section 1.3 (Figure 5.9.3). The upper 200 m is referred to as the photic or euphotic zone. This represents the region where enough light can penetrate to support photosynthesis, and it corresponds to the epipelagic zone. From 200-1000 m lies the dysphotic zone, or the twilight zone (corresponding with the mesopelagic zone). There is still some light at these depths, but not enough to support photosynthesis. Below 1000 m is the aphotic (or midnight) zone, where no light penetrates. This region includes the majority of the ocean volume, which exists in complete darkness.
