25 4.1 Beaches
For most people, when they think of coastal areas they picture a beach, and the beach that they imagine is probably a typical sandy beach composed of quartz sand grains. But beaches are comprised of whatever types of sediments are dominant in the local area. For example, parts of Hawaii and Iceland are famous for their black sand beaches, made up of eroded basalt and other volcanic materials. The beautiful tropical white sand beaches we see in travel ads are largely composed of the crushed calcium carbonate remains of coral skeletons (much of which has been chewed up and excreted by a fish before we happily run our toes through it!) Other beaches may lack sand altogether and instead be dominated by small shells, or larger rocks or pebbles (Figure 4.1.1).
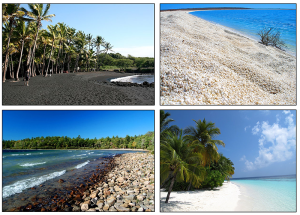
The shoreline is divided up into multiple zones (Figure 4.1.2). The backshore is the region of the beach above the high tide line, which is only submerged under unusually high wave conditions, such as during storms. The foreshore lies between the high tide and low tide lines; it is submerged during high tide and is exposed during low tide. The nearshore extends from the low tide line to the depth where wave action is no longer influenced by the bottom, i.e. to where the depth exceeds the wave base (section 3.1). Finally, the offshore zone represents the depths beyond the nearshore region.
Along the beach itself, the area above the high tide line is called the berm, which is usually dry and relatively flat. The berm often ends with a berm crest or berm scarp, which is a steeper wall carved out by wave action that leads down to the foreshore. The foreshore has a number of other names, including the beach face, the intertidal or littoral zone, and if the area is fairly flat, the low tide terrace. Just off shore from the beach there are often longshore bars and longshore troughs running parallel to the beach. The longshore bars are accumulations of sand that are deposited by wave action and longshore currents (section 4.2). The decrease in depth above longshore bars is what often causes waves to start to break well before reaching the beach (section 3.3).
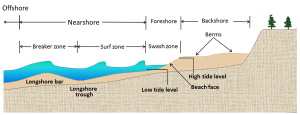
The sand or other particles that make up the beach are distributed by wave action. The water that moves over a beach through incoming waves is called swash, and it also contains suspended sand grains that can get deposited on the beach. Some of the swash percolates into the sand while the rest of the water washes back out as backwash as the wave recedes. Backwash removes sand from the beach and returns it to the ocean. Sand will therefore be deposited or eroded depending on which process is dominant. If wave action is light, a lot of incoming water gets absorbed by the sand, so swash dominates. Under heavier waves the beach becomes saturated with water, so less can be absorbed, and backwash is dominant. This leads to seasonal cycles in beach structure; waves are heavier during the winter as a result of stormier conditions at sea, so backwash dominates and sand is removed from the beach and deposited offshore in longshore bars. In the summer the waves are gentler, swash dominates, and the sand is transported from the longshore bar and deposited on the shore to create a wider, sandy beach (Figure 4.1.3).
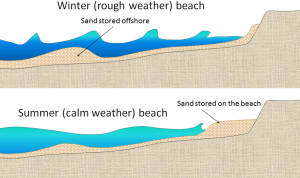
By Paul Webb, used under a CC-BY 4.0 international license. Download this book for free at https://rwu.pressbooks.pub/webboceanography/front-matter/preface/
Protecting the Marine Environment
Many national, state, and local governments and organizations have been struggling for decades to manage marine pollution and protect coastal environments. In the United States, the National Oceanographic and Atmospheric Administration (NOAA) overseas large portions of the coastal waters around North America, attempting to stop overfishing and restricting coastal development in many regions. Expanding efforts involve protecting coastal wetlands, mitigating coastal hazards, ensuring public coastal access, protecting beaches and coastal park lands, locating of energy and government facilities, and managing sensitive habitats, fishery areas, and aquaculture. Efforts are underway nationwide to prevent and control polluted runoff by replacing outdated storm water runoff and sewage systems, reducing agricultural pollutants, preventing or mitigating development within sensitive habitats and erosion-prone areas, and finding ways for communities to reduce refuse and debris from entering coastal waters.
Focus on Coral Reefs
Coral reefs (or coral ecosystems) are among the most important and also most sensitive habitats throughout the world’s oceans. Reefs provide habitat, spawning and nursery grounds for economically important fish species, and are hotspots of marine biodiversity. For humanity, coral reefs provide billions of dollars in economic and environmental benefits, including fishing, coastal protection, recreation, and tourism. Hundreds of millions of people worldwide depend of reef ecosystems for their livelihoods and food. However, coral ecosystems face serious threats from unsustainable fishing and land-based pollution (Figures 17-30 and 17-31).
Unfortunately, many of the world’s reefs have already been destroyed or severely damaged by pollution, unsustainable fishing practices, disease, introduction of invasive species, ship groundings, uncontrolled coastal development and other impacts.
Human activities are a primary cause for reef destruction. Pollutants from expanding coastal communities find their way to shallow coastal waters dominated by coral reefs, mostly in warm tropical waters. Many of the sea creatures, particularly invertebrates that attach to the seabed and filter seawater. Tourist visiting reefs step on fragile reef structures, introduce chemicals (such as zinc and other compounds in sun screen). Sewage and urban runoff carries silt (increasing turbidity) and introduce toxins that impact or kill reef organisms. Perhaps most alarming are the impacts of changes in water temperature and water chemistry associated with climate change.
Climate Change - The most important environmental issue of our times!
Studies conducted throughout the world’s oceans show the coral ecosystems are showing the detrimental effects of climate change caused by the burning of fossil fuels, deforestation, and bad agricultural practices that release large quantities of greenhouse gases into the atmosphere.
Climate change impacts coral ecosystems by increasing sea-surface temperatures and increased carbon dioxide levels in seawater. Long-term studies of CO2 concentrations in seawater show trends in reducing calcification rates in reef-building and reef-associated organisms. Increased sea surface temperature leads to coral bleaching (a result in the loss of symbiotic algae and bacteria) and death of skeletal reef-building organism. Weakened coral communities are susceptible to infection disease. Pollution from coastal development and agricultural runoff can also impede coral growth and reproduction, disrupt ecological functions, and cause disease.
Coal is the most carbon-intensive fossil fuel, producing the most carbon dioxide per unit volume burned. For every ton of coal burned, approximately 2.5 tons of CO2 is released into the air. Globally, coal is the largest-used fossil fuel source and the highest production of carbon dioxide emissions. Although coal represents only about one-third of share of fossil fuels consumed by the world’s total primary energy supply, coal is responsible for 43% of carbon dioxide emissions from burning fossil fuels.
Politics of Climate Change: Sadly, our world may be in big trouble because of the economics associated with energy and agricultural demands of a growing world population. Failure to address carbon emissions and the resultant impacts of rising temperatures and ocean acidification could make many marine and coastal management efforts futile. While reducing CO2 and other greenhouse gas emissions is vital to stabilize the global climate is essential, the excess that already exists in the atmosphere will persist throughout the next century.
Climate changes will have many impacts on marine systems including reduction in marine biodiversity, sea level is rising, and long-term forecasts predict changes in the frequency, intensity, and distribution of tropical storms as atmospheric and ocean circulation patterns change.
Politics vs. Technology: The key to saving or destroying our natural environments
The climate change issues will be every-increasingly important as the impacts become increasingly obvious as the number of natural and man-made disasters steadily rises. Humans have to collectively choose, through political means, to make the choices to change to cleaner technologies, and protecting and managing resources. The choices to move away from fossil-fuel consumption to alternative energy sources will be expensive and a hard fight because it will impact the livelihoods of many people (such as coal miners and workers in the petroleum industries). Predicted sea-level rise and global warming will impact all world's communities, both human and ecosystems. There will be many winners and losers in the transition to a cleaner, more sustainable world. Accepting the consequences for climate change will create many new jobs in the process. The longer humanity waits to make these changes, the greater the environmental problems will be in the future. "We can't fool mother nature!"
From Miracosta College, is shared under a not declared license and was authored, remixed, and/or curated by LibreTexts. Download this book for free at https://geo.libretexts.org/Bookshelves/Oceanography/Oceanography_101_(Miracosta)
Marine debris is a persistent pollution problem of global problem. Marine debris is a threat to wildlife, navigation safety, and is a factor affecting the economy and human health, particularly in poor countries in coastal regions. According to NOAA sources, approximately 1.4 billion pounds of trash per year ends up in the world’s oceans. The major components involve floating consumer plastic objects (including plastic bottles and caps, cigarette butts and lighters, and plastic bags), and these materials decay very slowly, if at all. Other materials include metals, rubber, paper, textiles, construction materials, and glass. The entire world’s oceans are impacted by solid wastes transported by currents. Large portions of the ocean in the center of the large gyres have become floating garbage patches where floating debris is accumulating.
Trash comes from many sources, mostly by careless acts that release trash into storm drains and eventually drain into coastal waterways. Some trash material blown into the by the wind. Much of it comes from coastal recreation and shoreline activities. In impoverished regions, garbage is intentionally dumped at sea. Fishing nets, hooks, lines, abandoned vessels are lost, drifting at sea. Trash washes up on beaches, accumulates on the seafloor, or drifts practically endlessly as sea. Trash is very harmful to wildlife. Small objects that are swallowed cannot be digested, often injuring or killing sea life. Bags, lines, and nets entangle animals, leading to starvation or strangulation.
Garbage patches are regions in the world's oceans where downwelling waters in the middle of ocean gyre region cause floating garbage to accumulate.
From Miracosta College, is shared under a not declared license and was authored, remixed, and/or curated by LibreTexts. Download this book for free at https://geo.libretexts.org/Bookshelves/Oceanography/Oceanography_101_(Miracosta)
The movement of surface currents also plays a role in the vertical movements of deeper water, mixing the upper water column. Upwelling is the process that brings deeper water to the surface, and its major significance is that it brings nutrient-rich deep water to the nutrient-deprived surface, stimulating primary production (see section 8.3). Downwelling is where surface water is forced downwards, where it may deliver oxygen to deeper water. Downwelling leads to reduced productivity, as it extends the depth of the nutrient-limited layer.
Upwelling occurs where surface currents are diverging, or moving away from each other. As the surface waters diverge, deeper water must be brought to the surface to replace it, creating upwelling zones. The upwelled water is cold and rich in nutrients, leading to high productivity. Many of the most productive regions on Earth are found in upwelling zones. In the equatorial Pacific, the trade winds blow the North and South Equatorial Currents towards the west, while Ekman transport causes the upper layers to move to the north and south in their respective hemispheres. This creates a divergence zone, and a region of upwelling and high productivity (Figure 7.5.1).
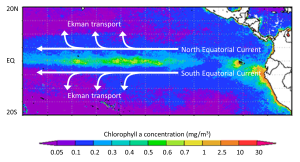
A similar process occurs near the Antarctic continent, creating one of the most productive regions on Earth, the Antarctic divergence. In this case, the West Wind Drift (Antarctic Circumpolar Current) is flowing parallel to, but in the opposite direction of the East Wind Drift. With both currents occurring in the Southern Hemisphere, Ekman transport will be to the left, so the eastward-flowing West Wind Drift water will be transported to the north, and the westward-flowing East Wind Drift water will be transported to the south, creating a highly productive divergence zone (Figure 7.5.2).

Downwelling occurs where surface currents converge. The converging water has nowhere to go but down, so the surface water sinks. Since surface water is usually low in nutrients, downwelling leads to low productivity zones. An example of a downwelling region is off of the Labrador coast in Canada, where the Gulf Stream, Labrador, and East Greenland Currents converge.
Coastal Upwelling
Upwelling and downwelling also occur along coasts, when winds move water towards or away from the coastline. Surface water moving away from land leads to upwelling, while downwelling occurs when surface water moves towards the land. Historically, some of the most productive commercial fishing grounds have been associated with coastal upwelling. Along the coast of California, the local prevailing winds blow towards the south. Ekman transport moves the surface layer 90o to the right of the wind, meaning the net Ekman transport is in an offshore direction. The water displaced near the coast is replaced by cold, nutrient-rich deeper water that is brought to the surface through upwelling, leading to high productivity (Figure 7.5.3).

The same process happens off of the coast of Peru, which for a long time had the world's largest commercial fishery. Winds along the Peruvian coast blow towards the north, and since Peru is in the Southern Hemisphere, the Ekman transport is 90o to the left of the wind, which causes the surface water to move offshore and leads to upwelling and productivity. In any coastal upwelling location, if the winds reverse, surface water moves towards the shore and downwelling is the result.
Upwelling can also occur due to geological features of the ocean floor. For example, as deep water currents encounter seamounts or other raised features, the water is forced upwards, bringing nutrient-rich water to the surface. This helps explain why productivity is often high in the water over seamounts.
Our modern understanding of tide formation stems from Isaac Newton's Law of Universal Gravitation, which states that any two objects have a gravitational attraction to each other. The magnitude of the force is proportional to the masses of the objects, and inversely proportional to the square of the distance between the objects, according to the equation in Figure 3.5.1.
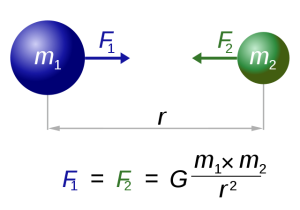
In the case of tides, there are a few other factors that modify this equation so that the distance (r) is cubed rather than squared, giving distance an even greater impact on tidal forces. But for our purposes, the important lesson is that the greater the masses of the objects, the greater the gravitational force, and the farther the objects are from each other, the weaker the force.
Such a gravitational force exists between the Earth and moon, attempting to pull them towards each other. Since the water covering Earth is fluid (unlike the solid land that is more resistant to tidal forces), this gravitational force pulls water towards the moon, creating a "bulge" of water on the side of the Earth facing the moon (Figure 3.5.2). This bulge always faces the moon, while the Earth rotates through it; the regions of Earth moving through the bulge experience a high tide, while those parts of the Earth away from the bulge experience a low tide.
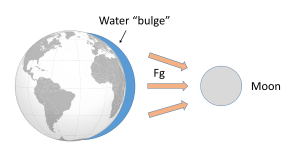
If the tides were this simple, everywhere on Earth would see one high tide per day, as there would only be a bulge of water on the side closest to the moon. However, if you have ever looked at tide charts, or lived near the ocean, you probably know that in most places there are two high tides and two low tides per day. Where is this second high tide "bulge" coming from?
The gravitational force between the Earth and moon might be expected to draw the two objects closer together, however, this is not happening. This is because the inward gravitational force is opposed by outward forces that keep the Earth and moon apart. The outward force is an intertial force created by the rotation of the Earth and moon. Contrary to popular belief, the moon is not simply rotating around the Earth; in fact, the Earth and moon are both rotating around each other. Imagine the Earth and moon as equal-sized objects revolving around a point at their center of mass. If both objects had the same mass, the center of rotation would be a point equidistant between the two objects. But since the mass of the Earth is 82 times greater than the mass of the moon, the center of revolution must be closer to the Earth. As an analogy, think about two people on a see-saw. If the people are of roughly equal size, they can sit on either end of the see-saw at it will rotate around a point at equal distance between them. But if the two people have very different masses, such as a large adult and a small child, the larger person must move closer to the pivot point for the see-saw to rotate effectively. In the same way, the center of rotation between the Earth and the moon (the barycenter) must be located closer to the Earth. In fact, the center of rotation lies within the Earth, about 1600 km below the surface. As the Earth and moon rotate around the barycenter, the moon travels much farther than the Earth, giving the impression that the moon is rotating around Earth (Figure 3.5.3).

The rotation of the Earth-moon system creates an outward inertial force, which balances the gravitational force to keep the two bodies in their orbits. The inertial force has the same magnitude everywhere on Earth, and is always directed away from the moon. Gravitational force, on the other hand, is always directed towards the moon, and is stronger on the side of the Earth closest to the moon. Figure 3.5.4 describes how these forces combine to create the tidal forces. At point O in the center of the Earth, the gravitational force (Fg) and the inertial force (Fr) are equal, and cancel each other out. On the side of Earth closest to the moon, the inward gravitational force (Fg) is greater than the outward inertial force (Fr); the net resulting force (A) is directed towards the moon, and creates a bulge of water on the side facing the moon. On the side of Earth opposite the moon, the outward inertial force is greater than the inward gravitational force; the net resulting force (C) is directed away from the moon, creating a water bulge directed away from the moon.
Now, as the Earth rotates through a 24 hour day, each region passes through two bulges, and experiences two high tides and two low tides per day. This represents Newton's Equilibrium Theory of Tides, where there are two high tides and two low tides per day, of similar heights, each six hours apart. But as with everything else in oceanography, reality is much more complex than this idealized situation.

Some of the additional complexity is because in addition to the moon, the sun also exerts tide-affecting forces on Earth. The solar gravitational and inertial forces arise for the same reasons described above for the moon, but the magnitudes of the forces are different. The sun is 27 million times more massive than the moon, but it is 387 times farther away from the Earth. Despite its larger mass, because the sun is so much farther away than the moon, the sun's gravitational forces are only about half as strong as the moon's (remember that distance is cubed in the gravity equation). The sun thus creates its own, smaller water bulges, independent of the moon's, that contribute to the creation of tides.
When the sun, Earth and moon are aligned, as occurs during new and full moons, the solar and lunar bulges are also aligned, and add to each other (constructive interference; see section 4.2) creating an especially high tidal range; high high tides and low low tides (Figure 3.5.5). This period of maximum tidal range is called a spring tide, and they occur every two weeks.

When the sun, Earth and moon are at 90o to each other, the solar and lunar bulges are out of phase, and cancel each other out (destructive interference). Now the tidal range is small, with low high tides and high low tides (Figure 3.5.6). These are neap tides, and occur every two weeks, when the moon is in its 1/4 and 3/4 phases (Figure 3.5.7).


By Paul Webb, used under a CC-BY 4.0 international license. Download this book for free at https://rwu.pressbooks.pub/webboceanography/front-matter/preface/
If one thing has been constant about Earth’s climate over geological time, it is its constant change. In the geological record, we can see this in the evidence of glaciations in the distant past, and we can also detect periods of extreme warmth by looking at the isotope composition of seafloor sediments. Not only has the climate changed frequently, the temperature fluctuations have been very significant. Today’s mean global temperature is about 15°C. However, during its coldest periods, the global mean was as cold as -50°C, while at various times during the Paleozoic and Mesozoic and during the Paleocene-Eocene thermal maximum, it was close to 30°C.
There are two parts to climate change, the first one is known as climate forcing, which is when conditions change to give the climate a little nudge in one direction or the other. The second part of climate change, and the one that typically does most of the work, is what we call a feedback. When a climate forcing changes the climate a little, a whole series of environmental changes take place, many of which either exaggerate the initial change (positive feedback), or suppress the change (negative feedback).
An example of a climate forcing mechanism is the increase in the amount of carbon dioxide (CO2) in the atmosphere that results from our use of fossil fuels. CO2 traps heat in the atmosphere and leads to climate warming. Warming changes vegetation patterns; contributes to the melting of snow, ice, and permafrost; causes sea level to rise; reduces the solubility of CO2 in sea water; and has a number of other minor effects. Most of these changes contribute to more warming. Melting of permafrost, for example, is a strong positive feedback because frozen soil contains trapped organic matter that is converted to CO2 and methane (CH4) when the soil thaws. Both these gases accumulate in the atmosphere and add to the warming effect. On the other hand, if warming causes more vegetation growth, that vegetation should absorb CO2, thus reducing the warming effect, which would be a negative feedback. Under our current conditions — a planet that still has lots of glacial ice and permafrost — most of the feedbacks that result from a warming climate are positive feedbacks and so the climate changes that we cause get naturally amplified by natural processes.
Natural Climate Forcing
Natural climate forcing has been going on throughout geological time. A wide range of processes has been operating at widely different time scales, from a few years to billions of years. The longest-term natural forcing variation is related to the evolution of the Sun. Like most other stars of a similar mass, our Sun is evolving. For the past 4.6 billion years, its rate of nuclear fusion has been increasing, and it is now emitting about 40% more energy (as light) than it did at the beginning of geological time. A difference of 40% is big, so it’s a little surprising that the temperature on Earth has remained at a reasonable and habitable temperature for all of this time. The mechanism for that relative climate stability has been the evolution of our atmosphere from one that was dominated by CO2, and also had significant levels of CH4 — both greenhouse gasses — to one with only a few hundred parts per million of CO2 and just under 1 part per million of CH4. Those changes to our atmosphere have been no accident; over geological time, life and its metabolic processes have evolved (such as the evolution of photosynthetic bacteria that consume CO2) and changed the atmosphere to conditions that remained cool enough to be habitable.
The position of the Earth relative to the Sun is another important component of natural climate forcing. Earth’s orbit around the Sun is nearly circular, but like all physical systems, it has natural oscillations. First, the shape of the orbit changes on a regular time scale (close to 100,000 years) from being close to circular to being very slightly elliptical. But the circularity of the orbit is not what matters; it is the fact that as the orbit becomes more elliptical, the position of the Sun within that ellipse becomes less central or more eccentric (Figure 6.5.1a). Eccentricity is important because when it is high, the Earth-Sun distance varies more from season to season than it does when eccentricity is low.
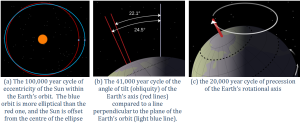
Second, Earth rotates around an axis through the North and South Poles, and that axis is at an angle to the plane of Earth’s orbit around the Sun (Figure 6.5.1b). The angle of tilt (also known as obliquity) varies on a time scale of 41,000 years. When the angle is at its maximum (24.5°), Earth’s seasonal differences are accentuated. When the angle is at its minimum (22.1°), seasonal differences are minimized. The current hypothesis is that glaciation is favored at low seasonal differences as summers would be cooler and snow would be less likely to melt and more likely to accumulate from year to year. Third, the direction in which Earth’s rotational axis points also varies, on a time scale of about 20,000 years (Figure 6.5.1c). This variation, known as precession, means that although the North Pole is presently pointing to the star Polaris (the pole star), in 10,000 years it will point to the star Vega. The importance of eccentricity, tilt, and precession to Earth’s climate cycles (now known as Milankovitch Cycles) was first pointed out by Yugoslavian engineer and mathematician Milutin Milankovitch in the early 1900s. Milankovitch recognized that although the variations in the orbital cycles did not affect the total amount of insolation (light energy from the Sun) that Earth received, it did affect where on Earth that energy was strongest.
Volcanic eruptions don’t just involve lava flows and exploding rock fragments; various particulates and gases are also released, the important ones being sulphur dioxide and CO2. Sulphur dioxide is an aerosol that reflects incoming solar radiation and has a net cooling effect that is short lived (a few years in most cases, as the particulates settle out of the atmosphere within a couple of years), and doesn’t typically contribute to longer-term climate change. Volcanic CO2 emissions can contribute to climate warming but only if a greater-than-average level of volcanism is sustained over a long time (at least tens of thousands of years). It is widely believed that the catastrophic end-Permian extinction (at 250 Ma) resulted from warming initiated by the eruption of the massive Siberian Traps over a period of at least a million years.
Ocean currents are important to climate, and currents also have a tendency to oscillate. Glacial ice cores show clear evidence of changes in the Gulf Stream that affected global climate on a time scale of about 1,500 years during the last glaciation. The east-west changes in sea-surface temperature and surface pressure in the equatorial Pacific Ocean, known as the El Niño Southern Oscillation or ENSO varies on a much shorter time scale of between two and seven years. These variations tend to garner the attention of the public because they have significant climate implications in many parts of the world. The strongest El Niños in recent decades were in 1983, 1998, and 2015 and those were very warm years from a global perspective. During a strong El Niño, the equatorial Pacific sea-surface temperatures are warmer than normal and heat the atmosphere above the ocean, which leads to warmer-than-average global temperatures.
Climate Feedbacks
As already stated, climate feedbacks are critically important in amplifying weak climate forcings into full-blown climate changes. Since Earth still has a very large volume of ice, mostly in the continental ice sheets of Antarctica and Greenland, but also in alpine glaciers and permafrost, melting is one of the key feedback mechanisms. Melting of ice and snow leads to several different types of feedbacks, an important one being a change in albedo, or the reflectivity of a surface. Earth’s various surfaces have widely differing albedos, expressed as the percentage of light that reflects off a given material. This is important because most solar energy that hits a very reflective surface is not absorbed and therefore does little to warm Earth. Water in the oceans or on a lake is one of the darkest surfaces, reflecting less than 10% of the incident light, while clouds and snow or ice are among the brightest surfaces, reflecting 70% to 90% of the incident light. When sea ice melts, as it has done in the Arctic Ocean at a disturbing rate over the past decade, the albedo of the area affected changes dramatically, from around 80% down to less than 10%. Much more solar energy is absorbed by the water than by the pre-existing ice, and the temperature increase is amplified. The same applies to ice and snow on land, but the difference in albedo is not as great. When ice and snow on land melt, sea level rises. (Sea level is also rising because the oceans are warming and that increases their volume). A higher sea level means a larger proportion of the planet is covered with water, and since water has a lower albedo than land, more heat is absorbed and the temperature goes up a little more. Since the last glaciation, sea-level rise has been about 125 m; a huge area that used to be land is now flooded by heat-absorbent seawater. During the current period of anthropogenic climate change, sea level has risen only about 20 cm, and although that doesn’t make a big change to albedo, sea-level rise is accelerating.
Most of northern Canada, Alaska, Russia, and Scandinavia has a layer of permafrost that ranges from a few centimeters to hundreds of meters in thickness. Permafrost is a mixture of soil and ice and it also contains a significant amount of trapped organic carbon that is released as CO2 and CH4 when the permafrost breaks down. Because the amount of carbon stored in permafrost is in the same order of magnitude as the amount released by burning fossil fuels, this is a feedback mechanism that has the potential to equal or surpass the forcing that has unleashed it. In some polar regions, including northern Canada, permafrost includes methane hydrate, a highly concentrated form of CH4 trapped in solid form. Breakdown of permafrost releases this CH4. Even larger reserves of methane hydrate exist on the seafloor, and while it would take significant warming of ocean water down to a depth of hundreds of meters, this too is likely to happen in the future if we don’t limit our impact on the climate. There is strong isotopic evidence that the Paleocene-Eocene thermal maximum was caused, at least in part, by a massive release of sea-floor methane hydrate.
There is about 45 times as much carbon in the ocean (as dissolved bicarbonate ions, HCO3-) as there is in the atmosphere (as CO2), and there is a steady exchange of carbon between the two reservoirs (see section 5.5). But the solubility of CO2 in water decreases as the temperature goes up. In other words, the warmer it gets, the more oceanic bicarbonate that gets transferred to the atmosphere as CO2. That makes CO2 solubility another positive feedback mechanism. Vegetation growth responds positively to both increased temperatures and elevated CO2 levels, and so in general, it represents a negative feedback to climate change because the more the vegetation grows, the more CO2 is taken from the atmosphere. But it’s not quite that simple, because when trees grow bigger and more vigorously, forests become darker (they have lower albedo) so they absorb more heat. Furthermore, climate warming isn’t necessarily good for vegetation growth; some areas have become too hot, too dry, or even too wet to support the plant community that was growing there, and it might take centuries for something to replace it successfully. All of these positive (and negative) feedbacks work both ways. For example, during climate cooling, growth of glaciers leads to higher albedos, and formation of permafrost results in storage of carbon that would otherwise have returned quickly to the atmosphere.
Anthropogenic Climate Change
When we talk about anthropogenic climate change, we are generally thinking of the industrial era, which really got going when we started using fossil fuels (coal to begin with, and later oil and natural gas) to drive machinery and trains, and to generate electricity. That was around the middle of the 18th century. The issue with fossil fuels is that they involve burning carbon that was naturally stored in the crust over hundreds of millions of years as part of Earth’s process of counteracting the warming Sun.
A rapidly rising population, the escalating level of industrialization and mechanization of our lives, and an increasing dependence on fossil fuels have driven the anthropogenic climate change of the past century. The trend of mean global temperatures since 1850 is shown in Figure 6.5.2. For approximately the past 55 years, the temperature has increased at a relatively steady and disturbingly rapid rate, especially compared to past changes. The average temperature now is approximately 1.1°C higher than before industrialization, and two-thirds of this warming has occurred since 1975.
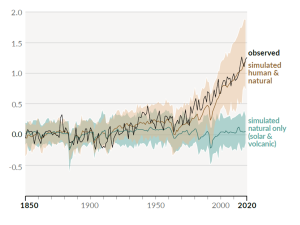
The Intergovernmental Panel on Climate Change (IPCC), established by the United Nations in 1988, is responsible for reviewing the scientific literature on climate change and issuing periodic reports on several topics, including the scientific basis for understanding climate change, our vulnerability to observed and predicted climate changes, and what we can do to limit climate change and minimize its impacts. Figure 6.5.3, from the sixth report of the IPCC, issued in preliminary form in 2021, shows the relative contributions of various greenhouse gases and other factors to current climate forcing, based on the changes from levels that existed in 1750.
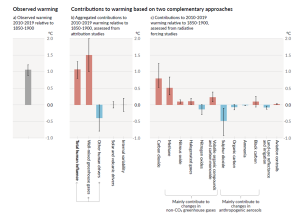
The biggest anthropogenic contributor to warming is the emission of CO2, which accounts for 50% of positive forcing. CH4 and its atmospheric derivatives (CO2, H2O, and O3) account for 29%, and the halocarbon gases (mostly leaked from air-conditioning appliances) and nitrous oxide (N2O) (from burning fossils fuels) account for 5% each. Carbon monoxide (CO) (also produced by burning fossil fuels) accounts for 7%, and the volatile organic compounds other than methane (NMVOC) account for 3%. CO2 emissions come mostly from coal- and gas-fired power stations, motorized vehicles (cars, trucks, and aircraft), and industrial operations (e.g., smelting), and indirectly from forestry. CH4 emissions come from production of fossil fuels (escape from coal mining and from gas and oil production), livestock farming (mostly beef), landfills, and wetland rice farming. N2O and CO come mostly from the combustion of fossil fuels. In summary, close to 70% of our current greenhouse gas emissions come from fossil fuel production and use, while most of the rest comes from agriculture and landfills. Figure 6.5.4 shows the IPCC’s projections for temperature increases over the next 100 years as a result of these increasing greenhouse gases.
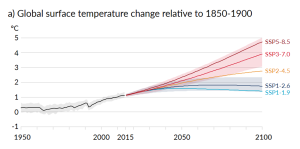
Impacts of Climate Change
We’ve all experienced the effects of climate change over the past decade. However, it’s not straightforward for climatologists to make the connection between a warming climate and specific weather events, and most are justifiably reluctant to ascribe any specific event to climate change. In this respect, the best measures of climate change are those that we can detect over several decades, such as the temperature changes shown in Figure 6.5.2, or the sea level rise shown in Figure 6.5.5. As already stated, sea level has risen approximately 20 cm since 1750, and that rise is attributed to both warming (and therefore expanding) seawater and melting glaciers and other land-based snow and ice (melting of sea ice does not contribute directly to sea level rise as it is already floating in the ocean).

Projections for sea level rise to the end of this century vary widely. This is in large part because we do not know which of the above climate change scenarios (Figure 6.5.4) we will most closely follow, but many are in the range from 0.5 m to 2.0 m. One of the problems in predicting sea level rise is that we do not have a strong understanding of how large ice sheets, such as Greenland and Antarctica, will respond to future warming. Another issue is that the oceans don’t respond immediately to warming. For example, with the current amount of warming, we are already committed to a future sea level rise of between 1.3 m and 1.9 m, even if we could stop climate change today. This is because it takes decades to centuries for the existing warming of the atmosphere to be transmitted to depth within the oceans and to exert its full impact on large glaciers. Most of that committed rise would take place over the next century, but some would be delayed longer. And for every decade that the current rates of climate change continue, that number increases by another 0.3 m. In other words, if we don’t make changes quickly, by the end of this century we’ll be locked into 3 m of future sea level rise. In a 2008 report, the Organization for Economic Co-operation and Development (OECD) estimated that by 2070 approximately 150 million people living in coastal areas could be at risk of flooding due to the combined effects of sea level rise, increased storm intensity, and land subsidence. The assets at risk (buildings, roads, bridges, ports, etc.) are in the order of $35 trillion ($35,000,000,000,000). Countries with the greatest exposure of population to flooding are China, India, Bangladesh, Vietnam, U.S.A., Japan, and Thailand. Some of the major cities at risk include Shanghai, Guangzhou, Mumbai, Kolkata, Dhaka, Ho Chi Minh City, Tokyo, Miami, and New York.
One of the other risks for coastal populations, besides sea level rise, is that climate warming is also associated with an increase in the intensity of tropical storms (e.g., hurricanes or typhoons; see section 6.4), which almost always bring serious flooding from intense rain and storm surges. Some recent examples are New Orleans in 2005 with Hurricane Katrina, and New Jersey and New York in 2012 with Hurricane Sandy. Tropical storms get their energy from the evaporation of warm seawater in tropical regions. In the Atlantic Ocean, this takes place between 8° and 20° N in the summer. Figure 6.5.6 shows the variations in the sea-surface temperature (SST) of the tropical Atlantic Ocean (in blue) versus the amount of power represented by Atlantic hurricanes between 1950 and 2008 (in red). Not only has the overall intensity of Atlantic hurricanes increased with the warming since 1975, but the correlation between hurricanes and sea-surface temperatures is very strong over that time period.

The geographical ranges of diseases and pests, especially those caused or transmitted by insects, have been shown to extend toward temperate regions because of climate change. West Nile virus and Lyme disease are two examples that already directly affect North Americans, while dengue fever could be an issue in the future (dengue became a "nationally notifiable condition" in the United States in 2010). For several weeks in July and August of 2010, a massive heat wave affected western Russia, especially the area southeast of Moscow, and scientists have stated that climate change was a contributing factor. Temperatures soared to over 40°C, as much as 12°C above normal over a wide area, and wildfires raged in many parts of the country. Over 55,000 deaths are attributed to the heat and to respiratory problems associated with the fires. A summary of the impacts of climate change on natural disasters is given in Figure 6.5.7. The major types of disasters related to climate are floods and storms, but the health implications of extreme temperatures are also becoming a great concern. In the decade 1971 to 1980, extreme temperatures were the fifth most common natural disasters; by 2001 to 2010, they were the third most common.

Most ocean waves are generated by wind. Wind blowing across the water's surface creates little disturbances called capillary waves, or ripples that start from gentle breezes (Figure 3.2.1). Capillary waves have a rounded crest with a V-shaped trough, and wavelengths less than 1.7 cm. These small ripples give the wind something to "grip" onto to generate larger waves when the wind energy increases, and once the wavelength exceeds 1.7 cm the wave transitions from a capillary wave to a wind wave. As waves are produced, they are opposed by a restoring force that attempts to return the water to its calm, equilibrium condition. The restoring force of the small capillary waves is surface tension, but for larger wind-generated waves gravity becomes the restoring force.
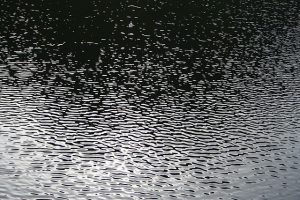
As the energy of the wind increases, so does the size, length and speed of the resulting waves. There are three important factors determining how much energy is transferred from wind to waves, and thus how large the waves will get:
- Wind speed.
- The duration of the wind, or how long the wind blows continuously over the water.
- The distance over which the wind blows across the water in the same direction, also known as the fetch.
Increasing any of these factors increases the energy of wind waves, and therefore their size and speed. But there is an upper limit to how large wind-generated waves can get. As wind energy increases, the waves receive more energy and they get both larger and steeper (recall from section 3.1 that wave steepness = height/wavelength). When the wave height exceeds 1/7 of the wavelength, the wave becomes unstable and collapses, forming whitecaps.
The ocean surface represents an irregular mixture of hundreds of waves of different speeds and sizes, all coming from different directions and interacting with each other. A histogram of wave heights within this mixture reveals a bell-shaped curve (Figure 3.2.2). In addition to basic statistics such as mode (most probable), median and mean wave height, wave heights are also reported in other ways. Marine weather forecasts and ship and buoy data often report significant wave height (Hs), which is the mean height of the largest one-third of the waves. Mean wave height is approximately equal to two-thirds of the significant wave height. Finally, there is the minimum height of the highest 10% of waves (the 90th percentile of wave heights), often expressed as H1/10.
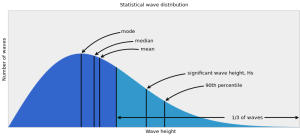
Under strong wind conditions, the ocean surface becomes a chaotic mixture of choppy, whitecapped wind-generated waves. The term sea state describes the size and extent of the wind-generated waves in a particular area. When the waves are at their maximum size for the existing wind speed, duration, and fetch, it is referred to as a fully developed sea. The sea state is often reported on the Beaufort scale, ranging from 0-12, where 0 means calm, windless and waveless conditions, while Beaufort 12 is a hurricane (see box below).
The Beaufort Scale
The Beaufort scale is used to describe the wind and sea state conditions on the ocean. It is an observational scale based on the judgement of the observer, rather than one dictated by accurate measurements of wave height. Beaufort 0 represents calm, flat conditions, while Beaufort 12 represents a hurricane.
(Images by United States National Weather Service (http://www.crh.noaa.gov/mkx/marinefcst.php) [Public domain], via Wikimedia Commons).
A fully developed sea often occurs under stormy conditions, where high winds create a chaotic, random pattern of waves and whitecaps of varying sizes. The waves will propagate outwards from the center of the storm, powered by the strong winds. However, as the storm subsides and the winds weaken, these irregular seas will sort themselves out into more ordered patterns. Recall that open ocean waves will usually be deep water waves, and their speed will depend on their wavelength (section 3.1). As the waves move away from the storm center, they sort themselves out based on speed, with longer wavelength waves traveling faster than shorter wavelength waves. This means that eventually all of the waves in a particular area will be traveling with the same wavelength, creating regular, long period waves called swell (Figure 3.2.3). We experience swell as the slow up and down or rocking motion we feel on a boat, or with the regular arrival of waves on shore. Swell can travel very long distances without losing much energy, so we can observe large swells arriving at the shore even where there is no local wind; the waves were produced by a storm far offshore, and were sorted into swell as they traveled towards the coast.
Figure 3.2.3 Ocean swell, the regular pattern of waves of equal wavelength (Phillip Capper [CC BY 2.0], via Wikimedia Commons).
Because swell travels such long distances, eventually swells coming from different directions will run into each other, and when they do they create interference patterns. The interference pattern is created by adding the features of the waves together, and the type of interference that is created depends on how the waves interact with each other (Figure 3.2.4). Constructive interference occurs when the two waves are completely in phase; the crest of one wave lines up exactly with the crest of the other wave, as do the troughs of the two waves. Adding the two crest together creates a crest that is higher than in either of the source waves, and adding the troughs creates a deeper trough than in the original waves. The result of constructive interference is therefore to create waves that are larger than the original source waves. In destructive interference, the waves interact completely out of phase, where the crest of one wave aligns with the trough of the other wave. In this case, the crest and the trough work to cancel each other out, creating a wave that is smaller than either of the source waves. In reality, it is rare to find perfect constructive or destructive interference as displayed in Figure 3.2.4. Most interference by swells at sea is mixed interference, which contains a mix of both constructive and destructive interference. The interacting swells do not have the same wavelength, so some points show constructive interference, and some points show destructive interference, to varying degrees. This results in an irregular pattern of both small and large waves, called surf beat.
It is important to point out that these interference patterns are only temporary disturbances, and do not affect the properties of the source waves. Moving swells interact and create interference where they meet, but each wave continues on unaffected after the swells pass each other.

About half of the waves in the open sea are less than 2 m high, and only 10-15% exceed 6 m. But the ocean can produce some extremely large waves. The largest wind wave reliably measured at sea occurred in the Pacific Ocean in 1935, and was measured by the navy tanker the USS Ramapo. Its crew measured a wave of 34 m or about 112 ft high! Occasionally constructive interference will produce waves that are exceptionally large, even when all of the surrounding waves are of normal height. These random, large waves are called rogue waves (Figure 3.2.5). A rogue wave is usually defined as a wave that is at least twice the size of the significant wave height, which is the average height of the highest one-third of waves in the region. It is not uncommon for rogue waves to reach heights of 20 m or more.
Figure 3.2.5 A rogue wave in the Bay of Biscay, off of the French coast, ca. 1940 (NOAA, [Public domain], via Wikimedia Commons).
Rogue waves are particularly common off of the southeast coast of South Africa, a region referred to as the "wild coast." Here, Antarctic storm waves move north into the oncoming Agulhas Current, and the wave energy gets focused over a narrow area, leading to constructive interference. This area may be responsible for sinking more ships than anywhere else on Earth. On average about 100 ships are lost every year across the globe, and many of these losses are probably due to rogue waves.
Waves in the Southern Ocean are generally fairly large (the red areas in Figure 3.2.6) because of the strong winds and the lack of landmasses, which provide the winds with a very long fetch, allowing them to blow unimpeded over the ocean for very long distances. These latitudes have been termed the “Roaring Forties”, “Furious Fifties”, and “Screaming Sixties” due to the high winds.

Most ocean waves are generated by wind. Wind blowing across the water's surface creates little disturbances called capillary waves, or ripples that start from gentle breezes (Figure 3.2.1). Capillary waves have a rounded crest with a V-shaped trough, and wavelengths less than 1.7 cm. These small ripples give the wind something to "grip" onto to generate larger waves when the wind energy increases, and once the wavelength exceeds 1.7 cm the wave transitions from a capillary wave to a wind wave. As waves are produced, they are opposed by a restoring force that attempts to return the water to its calm, equilibrium condition. The restoring force of the small capillary waves is surface tension, but for larger wind-generated waves gravity becomes the restoring force.
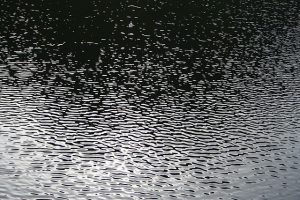
As the energy of the wind increases, so does the size, length and speed of the resulting waves. There are three important factors determining how much energy is transferred from wind to waves, and thus how large the waves will get:
- Wind speed.
- The duration of the wind, or how long the wind blows continuously over the water.
- The distance over which the wind blows across the water in the same direction, also known as the fetch.
Increasing any of these factors increases the energy of wind waves, and therefore their size and speed. But there is an upper limit to how large wind-generated waves can get. As wind energy increases, the waves receive more energy and they get both larger and steeper (recall from section 3.1 that wave steepness = height/wavelength). When the wave height exceeds 1/7 of the wavelength, the wave becomes unstable and collapses, forming whitecaps.
The ocean surface represents an irregular mixture of hundreds of waves of different speeds and sizes, all coming from different directions and interacting with each other. A histogram of wave heights within this mixture reveals a bell-shaped curve (Figure 3.2.2). In addition to basic statistics such as mode (most probable), median and mean wave height, wave heights are also reported in other ways. Marine weather forecasts and ship and buoy data often report significant wave height (Hs), which is the mean height of the largest one-third of the waves. Mean wave height is approximately equal to two-thirds of the significant wave height. Finally, there is the minimum height of the highest 10% of waves (the 90th percentile of wave heights), often expressed as H1/10.
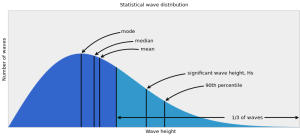
Under strong wind conditions, the ocean surface becomes a chaotic mixture of choppy, whitecapped wind-generated waves. The term sea state describes the size and extent of the wind-generated waves in a particular area. When the waves are at their maximum size for the existing wind speed, duration, and fetch, it is referred to as a fully developed sea. The sea state is often reported on the Beaufort scale, ranging from 0-12, where 0 means calm, windless and waveless conditions, while Beaufort 12 is a hurricane (see box below).
The Beaufort Scale
The Beaufort scale is used to describe the wind and sea state conditions on the ocean. It is an observational scale based on the judgement of the observer, rather than one dictated by accurate measurements of wave height. Beaufort 0 represents calm, flat conditions, while Beaufort 12 represents a hurricane.
(Images by United States National Weather Service (http://www.crh.noaa.gov/mkx/marinefcst.php) [Public domain], via Wikimedia Commons).
A fully developed sea often occurs under stormy conditions, where high winds create a chaotic, random pattern of waves and whitecaps of varying sizes. The waves will propagate outwards from the center of the storm, powered by the strong winds. However, as the storm subsides and the winds weaken, these irregular seas will sort themselves out into more ordered patterns. Recall that open ocean waves will usually be deep water waves, and their speed will depend on their wavelength (section 3.1). As the waves move away from the storm center, they sort themselves out based on speed, with longer wavelength waves traveling faster than shorter wavelength waves. This means that eventually all of the waves in a particular area will be traveling with the same wavelength, creating regular, long period waves called swell (Figure 3.2.3). We experience swell as the slow up and down or rocking motion we feel on a boat, or with the regular arrival of waves on shore. Swell can travel very long distances without losing much energy, so we can observe large swells arriving at the shore even where there is no local wind; the waves were produced by a storm far offshore, and were sorted into swell as they traveled towards the coast.
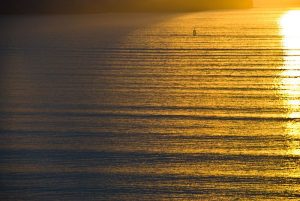
Because swell travels such long distances, eventually swells coming from different directions will run into each other, and when they do they create interference patterns. The interference pattern is created by adding the features of the waves together, and the type of interference that is created depends on how the waves interact with each other (Figure 3.2.4). Constructive interference occurs when the two waves are completely in phase; the crest of one wave lines up exactly with the crest of the other wave, as do the troughs of the two waves. Adding the two crest together creates a crest that is higher than in either of the source waves, and adding the troughs creates a deeper trough than in the original waves. The result of constructive interference is therefore to create waves that are larger than the original source waves. In destructive interference, the waves interact completely out of phase, where the crest of one wave aligns with the trough of the other wave. In this case, the crest and the trough work to cancel each other out, creating a wave that is smaller than either of the source waves. In reality, it is rare to find perfect constructive or destructive interference as displayed in Figure 3.2.4. Most interference by swells at sea is mixed interference, which contains a mix of both constructive and destructive interference. The interacting swells do not have the same wavelength, so some points show constructive interference, and some points show destructive interference, to varying degrees. This results in an irregular pattern of both small and large waves, called surf beat.
It is important to point out that these interference patterns are only temporary disturbances, and do not affect the properties of the source waves. Moving swells interact and create interference where they meet, but each wave continues on unaffected after the swells pass each other.

About half of the waves in the open sea are less than 2 m high, and only 10-15% exceed 6 m. But the ocean can produce some extremely large waves. The largest wind wave reliably measured at sea occurred in the Pacific Ocean in 1935, and was measured by the navy tanker the USS Ramapo. Its crew measured a wave of 34 m or about 112 ft high! Occasionally constructive interference will produce waves that are exceptionally large, even when all of the surrounding waves are of normal height. These random, large waves are called rogue waves (Figure 3.2.5). A rogue wave is usually defined as a wave that is at least twice the size of the significant wave height, which is the average height of the highest one-third of waves in the region. It is not uncommon for rogue waves to reach heights of 20 m or more.
Figure 3.2.5 A rogue wave in the Bay of Biscay, off of the French coast, ca. 1940 (NOAA, [Public domain], via Wikimedia Commons).
Rogue waves are particularly common off of the southeast coast of South Africa, a region referred to as the "wild coast." Here, Antarctic storm waves move north into the oncoming Agulhas Current, and the wave energy gets focused over a narrow area, leading to constructive interference. This area may be responsible for sinking more ships than anywhere else on Earth. On average about 100 ships are lost every year across the globe, and many of these losses are probably due to rogue waves.
Waves in the Southern Ocean are generally fairly large (the red areas in Figure 3.2.6) because of the strong winds and the lack of landmasses, which provide the winds with a very long fetch, allowing them to blow unimpeded over the ocean for very long distances. These latitudes have been termed the “Roaring Forties”, “Furious Fifties”, and “Screaming Sixties” due to the high winds.

Mercury, lead, chromium, copper, cadmium, and arsenic are perhaps most significant. Mercury and lead are released into the environment from burning coal and fossil fuels, and by mining operations. Consumer products that contain lead, mercury, and other toxic metals end up in landfills which can leak into waterways. Lead from improperly disposed of batteries is a major contributor to lead contamination. Starting in the 1920‘s lead was used as an “additive” to gasoline to increase engine performance, but was found to be a toxic addition to air and soil pollution. It has been banned in most countries, but the lead is still finding its way into ocean waters. Lead and many other toxic compounds were also used in paints before being banned.
Mercury was used in gold mining and extraction, and in light bulbs, and used in many other industrial purposes, now mostly banned. Large quantities of mercury was mined in the New Almaden mercury mining district near San Jose, California. Over 65 million pound of mercury were extracted from the mines in the, nearly all of it was consumed in the gold fields along the western Sierra Mountains of central California and is a major contaminant in the Sacramento River system feeding into San Francisco Bay. Mercury is problematic in that it is concentrated in organisms highest in the food chain. In many areas, fish, such as tuna, have unsafe levels of mercury that, if over-consumed, can lead to mercury poisoning in humans. After WWII, a chemical process used to manufacture plastics released large quantities of mercury compounds into Minamoto Bay, Japan. The bio-accumulated mercury in fish that was the primary source of protein in the fishing community populations around the bay, nearly the entire population was sickened, many displaying severe neurological disorders.
Cadmium is used in non-rechargeable nickel-cadmium batteries, and is a major toxin in landfill waste waters. Both cadmium and arsenic are released by runoff from poorly managed mining operations, both past and present throughout the world today. Arsenic in a common natural contaminant in groundwater in some coastal regions. Various cancers and other diseases are linked to consuming contaminated water and food with high levels of arsenic.
Heavy metal pollutant come from many sources including mining, smelting, abandoned lead batteries and garbage, chromium from tanneries, and other local industrial sources mostly in impoverished urbanized areas where there is little or no regulation oversight. Modern electronic gadgets are host to many toxic metals and organometallic compounds and should be recycled (not thrown into landfills that may leak toxins). Research by world health organizations report that at least 100 million people worldwide suffer health effects or die from heavy-metal pollution each year.
From Miracosta College, is shared under a not declared license and was authored, remixed, and/or curated by LibreTexts. Download this book for free at https://geo.libretexts.org/Bookshelves/Oceanography/Oceanography_101_(Miracosta)