24 3.7 Tide Classification
With so many variables playing a role in the production of tides, it is understandable that not every place on Earth will experience exactly the same tidal conditions. There are three primary classifications for tides, depending on the number and relative heights of tidal cycles per day.
A diurnal tide consists of only one high tide and one low tide per day (Figure 3.7.1). “Diurnal” refers to a daily occurrence, so a situation where there is only one complete tidal cycle per day is considered a diurnal tide. Diurnal tides are common in the Gulf of Mexico, along the west coast of Alaska, and in parts of Southeast Asia.
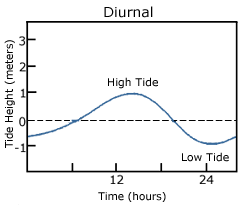
A semidiurnal tide exhibits two high and two low tides each day, with both highs and both lows of toughly equal height (Figure 3.7.2). “Semidiurnal” means “half of a day”; one tidal cycle takes half of a day, therefore there are two complete cycles per day. Semidiurnal tides are common along the east coasts of North America and Australia, the west coast of Africa, and most of Europe.
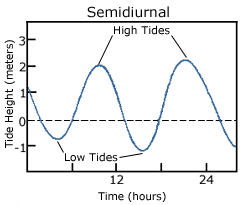
Mixed semidiurnal tides (or mixed tides), have two high tides and two low tides per day, but the heights of each tide differs; the two high tides are of different heights, as are the two low tides (Figure 3.7.3). The differences in height may be the result of amphidromic circulation, the angle of the moon, or any of the other variables discussed in section 3.6. Mixed semidiurnal tides are found along the Pacific coast of North America.
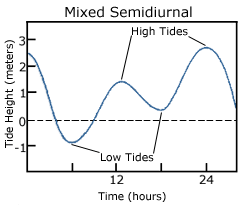
Figure 3.7.4 shows the distribution of the various tide types throughout the world.
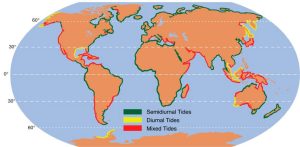
Tidal Currents
The movement of water with the rising and falling tide creates tidal currents. As the tide rises, water flows into an area, creating a flood current. As the tide falls and water flows out an ebb current is created. Slack water, or slack tides occur during the transition between incoming high and outgoing low tides, when there is no net water movement.
The strength of a tidal current depends on the volume of water that enters and exits with each tidal cycle (the tidal volume or tidal prism), and the area through which the water flows. A large tidal volume moving through a large area may create only a weak tidal current, as the volume is spread over a wide area. On the other hand, a narrow area may produce a strong tidal current even if the tidal volume is small, as all of the water is forced through a small area. It follows that the strongest tidal currents will result from a large tidal range moving through a narrow area.
Tidal bores occur where rivers meet the ocean. If the incoming tidal current is stronger than the river outflow, the tidal bore appears as a wave, or moving wall of water that moves up the river as the tide comes in (Figure 3.7.5).
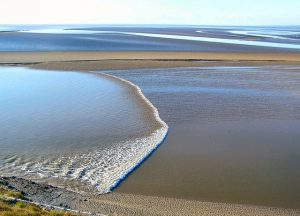
In many cases these tidal bores may move through a river or inlet for many kilometers, and if they are large enough they can form continually breaking waves that surfers can ride much farther and longer than a traditional ocean wave, such as the Severn Bore in England, shown in the video below.
Additional links for more information
- For an even more dramatic tidal bore, watch this video of the “Silver Dragon” on China’s Qiantang River
By Paul Webb, used under a CC-BY 4.0 international license. Download this book for free at https://rwu.pressbooks.pub/webboceanography/front-matter/preface/
Tsunamis loom large in popular culture, but there are a number of misconceptions about these large waves. First, tsunamis have nothing to do with the tides, so it is a misnomer to refer to them as "tidal waves." There are actual tidal waves (see section 3.5), but they are not related to tsunamis. Second, the giant, curling wave that is taller than skyscrapers and destroys cities in science fiction movies is also a fabrication, as tsunamis do not behave that way, as described below.
Tsunamis are large waves that are usually the result of seismic activity, such as the rising or falling of the seafloor due to earthquakes, although volcanic activity and landslides can also cause tsunamis in the form of splash waves (see section 3.1). As the seafloor rises or falls, so does the water column above it, creating waves. Only vertical seismic disturbances cause tsunamis, not horizontal movements. These vertical seafloor movements are usually less than 10 m high, so the resulting wave will be of an equal or lesser height at sea. While the tsunamis have a relatively small height at the point of origin, they have very long wavelengths (100-200 km). Because of the long wavelength, they behave as shallow water waves throughout the entire ocean; the depth of the ocean is always shallower than half of their wavelength. As shallow water waves, their speed depends on water depth, but they can still travel at speeds over 750 km/hr (Figure 3.4.1)!

When tsunamis approach land, they behave just like any other wave; as the depth becomes shallower, the waves slow down and the wave height begins to increase. However, contrary to popular belief, tsunamis do not arrive on shore as giant, cresting waves. Since their wavelength is so long, it is impossible for their height to ever exceed 1/7 of their wavelength, so the waves don’t actually curl or break. Instead, they usually hit the shore as sudden surges of water causing a very rapid increase in sea level, like that of an enormous rise in tide. It may take several minutes for the wave to pass, during which time sea level can rise to 40 m higher than usual.
Large tsunamis occur every 2-3 years, with very large, damaging events happening every 15-20 years. The most devastating tsunami in terms of loss of life resulted from a magnitude 9 earthquake in Indonesia in 2004 (Figure 3.4.2), which created waves up to 33 m tall and left about 230,000 people dead in Indonesia, Thailand, and Sri Lanka. In 2011 a 9.0 magnitude earthquake in Japan triggered a tsunami up to 40.5 m high, which resulted in over 18,000 deaths. This earthquake also caused the Fukishima nuclear accident, and moved Japan about 8 inches closer to the U.S.

By Paul Webb, used under a CC-BY 4.0 international license. Download this book for free at https://rwu.pressbooks.pub/webboceanography/front-matter/preface/
The primary surface current along the east coast of the United States is the Gulf Stream, which was first mapped by Benjamin Franklin in the 18th century (Figure 7.2.1). As a strong, fast current, it reduced the sailing time for ships traveling from the United States back to Europe, so sailors would use thermometers to locate its warm water and stay within the current.
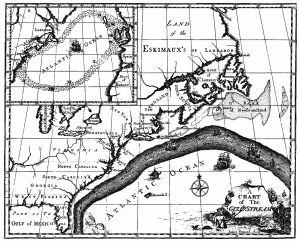
The Gulf Stream is formed from the convergence of the North Atlantic Equatorial Current bringing tropical water from the east, and the Florida Current that brings warm water from the Gulf of Mexico. The Gulf Stream takes this warm water and transports it northwards along the U.S. east coast (Figure 7.2.2). As a western boundary current, the Gulf Stream experiences western intensification (section 7.4), making the current narrow (50-100 km wide), deep (to depths of 1.5 km) and fast. With an average speed of 6.4 km/hr, and a maximum speed of about 9 km/hr, it is the fastest current in the world ocean. It also transports huge amounts of water, more than 100 times greater than the combined flow of all of the rivers on Earth.
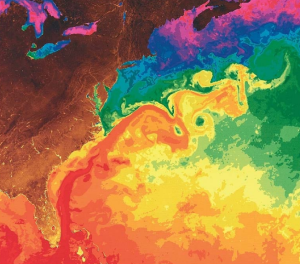
As the Gulf Stream approaches Canada, the current becomes wider and slower as the flow dissipates and it encounters the cold Labrador Current moving in from the north. At this point, the current begins to meander, or change from a fast, straight flow to a slower, looping current (Figure 7.2.2). Often these meanders loop so much that they pinch off and form large rotating water masses called rings or eddies, that separate from the Gulf Stream. If an eddy pinches off from the north side of the Gulf Stream, it entraps a mass of warm water and moves it north into the surrounding cold water of the North Atlantic. These warm core rings are shallow, bowl-shaped water masses about 1 km deep, and about 100 km across, that rotate clockwise as they carry warm water in to the North Atlantic (Figure 7.2.3). If the meanders pinch off at the southern boundary of the Gulf Stream, they form cold core rings that rotate counterclockwise and move to the south. Cold core rings are cone-shaped water masses extending down to over 3.5 km deep, and may be over 500 km wide at the surface.
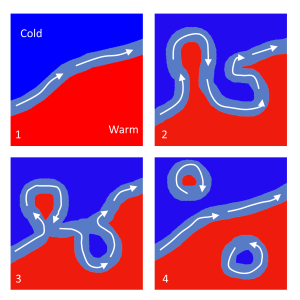
After the Gulf Stream meets the cold Labrador Current, it joins the North Atlantic Current, which transports the warm water towards Europe, where it moderates the European climate. It is estimated that Northern Europe is up to 9o C warmer than expected because of the Gulf Stream, and the warm water helps to keep many northern European ports ice-free in the winter.
In the east, the Gulf Stream merges into the Sargasso Sea, which is the area of the ocean within the rotation center of the North Atlantic gyre. The Sargasso Sea gets its name from the large floating mats of the marine algae Sargassum that are abundant on the surface (Figure 7.2.4). These Sargassum mats may play an important role in the early life stages of sea turtles, who may live and feed within the algae for many years before reaching adulthood.
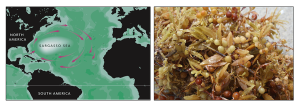
Pfeiffer Beach, California 2023 (Photo credit: Cristina Cardona)
This book is a collection of Open Educational Resources adapted and edited by Dr. Cristina Cardona, Associate Professor of Physical Sciences at CCBC Essex.
Chapter 10: Marine Vertebrates, sections 10.2-10.6 were written by Dr. Cristina Cardona, CCBC Essex.
Contributing authors:
- Paul Webb, Roger Williams University
- Miracosta College faculty
- University of California, Davis (Hill et al.)
- University of California, Davis (Keddy)
- Steven Earle
Oxygen and carbon dioxide are involved in the same biological processes in the ocean, but in opposite ways; photosynthesis consumes CO2 and produces O2, while respiration and decomposition consume O2 and produce CO2. Therefore it should not be surprising that oceanic CO2 profiles are essentially the opposite of dissolved oxygen profiles (Figure 5.5.1). At the surface, photosynthesis consumes CO2 so CO2 levels remain relatively low. In addition, organisms that utilize carbonate in their shells are common near the surface, further reducing the amount of dissolved CO2.
In deeper water, CO2 concentration increases as respiration exceeds photosynthesis, and decomposition of organic matter adds additional CO2 to the water. As with oxygen, there is often more CO2 at depth because cold bottom water holds more dissolved gases, and high pressures increase solubility. Deep water in the Pacific contains more CO2 than the Atlantic as the Pacific water is older and has accumulated more CO2 from the respiration of benthic organisms.
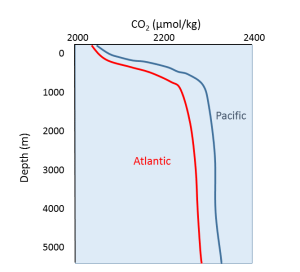
But the behavior of carbon dioxide in the ocean is more complex than the figure above would suggest. When CO2 gas dissolves in the ocean, it interacts with the water to produce a number of different compounds according to the reaction below:
CO2 + H2O ↔ H2CO3 ↔ H+ + HCO3- ↔ 2H+ + CO32-
CO2 reacts with water to produce carbonic acid (H2CO3), which then dissociates into bicarbonate (HCO3-) and hydrogen ions (H+). The bicarbonate ions can further dissociate into carbonate (CO32-) and additional hydrogen ions (Figure 5.5.2).
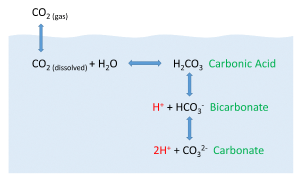
Most of the CO2 dissolving or produced in the ocean is quickly converted to bicarbonate. Bicarbonate accounts for about 92% of the CO2 dissolved in the ocean, and carbonate represents around 7%, so only about 1% remains as CO2, and little gets absorbed back into the air. The rapid conversion of CO2 into other forms prevents it from reaching equilibrium with the atmosphere, and in this way, water can hold 50-60 times as much CO2 and its derivatives as the air.
CO2 and pH
The equation above also illustrates carbon dioxide's role as a buffer, regulating the pH of the ocean. Recall that pH reflects the acidity or basicity of a solution. The pH scale runs from 0-14, with 0 indicating a very strong acid, and 14 representing highly basic conditions. A solution with a pH of 7 is considered neutral, as is the case for pure water. The pH value is calculated as the negative logarithm of the hydrogen ion concentration according to the equation:
pH = -log10[H+]
Therefore, a high concentration of H+ ions leads to a low pH and acidic condition, while a low H+ concentration indicates a high pH and basic conditions. It should also be noted that pH is described on a logarithmic scale, so every one point change on the pH scale actually represents an order of magnitude (10 x) change in solution strength. So a pH of 6 is 10 times more acidic than a pH of 7, and a pH of 5 is 100 times (10 x 10) more acidic than a pH of 7.
Carbon dioxide and the other carbon compounds listed above play an important role in buffering the pH of the ocean. Currently, the average pH for the global ocean is about 8.1, meaning seawater is slightly basic. Because most of the inorganic carbon dissolved in the ocean exists in the form of bicarbonate, bicarbonate can respond to disturbances in pH by releasing or incorporating hydrogen ions into the various carbon compounds. If pH rises (low [H+]), bicarbonate may dissociate into carbonate, and release more H+ ions, thus lowering pH. Conversely, if pH gets too low (high [H+]), bicarbonate and carbonate may incorporate some of those H+ ions and produce bicarbonate, carbonic acid, or CO2 to remove H+ ions and raise the pH. By shuttling H+ ions back and forth between the various compounds in this equation, the pH of the ocean is regulated and conditions remain favorable for life.
CO2 and Ocean Acidification
In recent years there has been rising concern about the phenomenon of ocean acidification. As described in the processes above, the addition of CO2 to seawater lowers the pH of the water. As anthropogenic sources of atmospheric CO2 have increased since the Industrial Revolution, the oceans have been absorbing an increasing amount of CO2, and researchers have documented a decline in ocean pH from about 8.2 to 8.1 in the last century. This may not appear to be much of a change, but remember that since pH is on a logarithmic scale, this decline represents a 30% increase in acidity. It should be noted that even at a pH of 8.1 the ocean is not actually acidic; the term "acidification" refers to the fact that the pH is becoming lower, i.e. the water is moving towards more acidic conditions.
Figure 5.5.3 presents data from observation stations in and around the Hawaiian Islands. As atmospheric levels of CO2 have increased, the CO2 content of the ocean water has also increased, leading to a reduction in seawater pH. Some models suggest that at the current rate of CO2 addition to the atmosphere, by 2100 ocean pH may be further reduced to around 7.8, which would represent more than a 120% increase in ocean acidity since the Industrial Revolution.
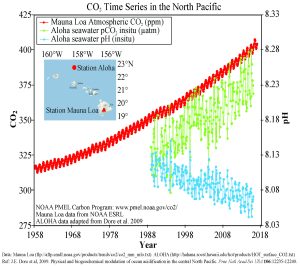
Why is this important? Declining pH can impact many biological systems. Of particular concern are organisms that secrete calcium carbonate shells or skeletons, such as corals, shellfish, and may planktonic organisms. At lower pH levels, calcium carbonate dissolves, eroding the shells and skeletons of these organisms (Figure 5.5.4).
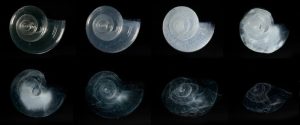
Not only does a declining pH lead to increased rates of dissolution of calcium carbonate, it also diminishes the amount of free carbonate ions in the water. The relative proportions of the different carbon compounds in seawater is dependent on pH (Figure 5.5.6). As pH declines, the amount of carbonate declines, so there is less available for organisms to incorporate into their shells and skeletons. So ocean acidification both dissolves existing shells and makes it harder for shell formation to occur.

Additional links for more information:
- NOAA Ocean Acidification Program website http://oceanacidification.noaa.gov/