5 1.5 Structure of Earth
Materials in the early Earth were sorted through the process of differentiation, with denser materials like iron and nickel sinking to the center, and lighter materials (oxygen, silicon, magnesium) remaining near the surface. As a result, the Earth is composed of layers of different composition and increasing density as you move from the surface to the center (Figure 1.5.1).
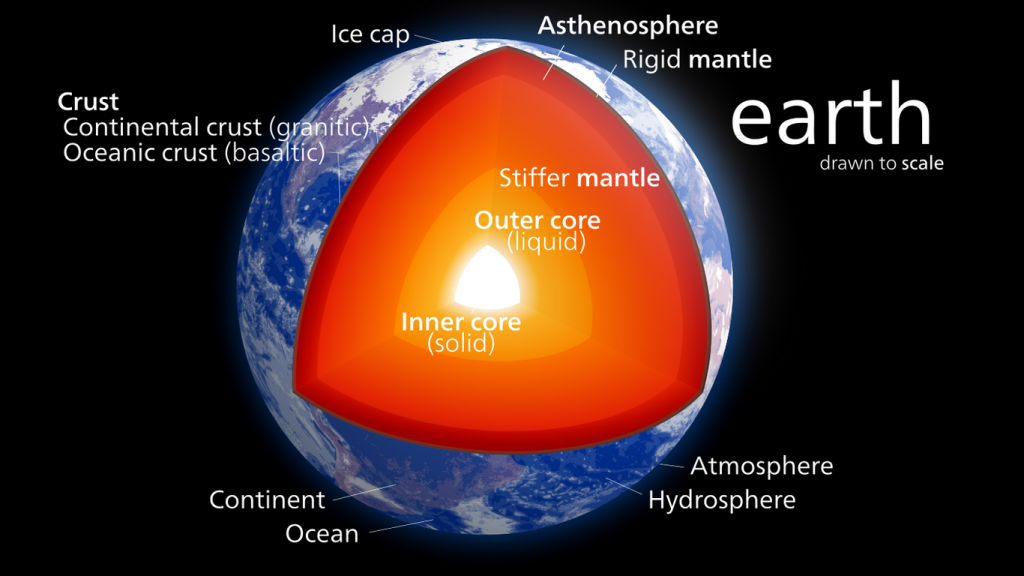
The traditional view based on chemical composition recognizes four distinct layers:
The inner core lies at the center of the Earth, and is about 1200 km thick. It is composed primarily of iron alloys and nickel, with about 10% comprised of oxygen, sulfur or hydrogen. The temperature in the inner core is about 6000 oC (10,800 oF), which is roughly the temperature of the surface of the sun. Despite the high temperature that should melt these metals, the extreme pressure (from literally the weight of the world) keeps the inner core in the solid phase. The solid metals also make the inner core very dense, at about 17 g/cm3, giving the inner core about one-third of the Earth’s total mass.
The outer core sits outside of the inner core. It has the same composition as the inner core, but it exists as a fluid, rather than a solid. The temperature is 4000-6000 oC, and the metals remain in the liquid state because the pressure is not as great as in the inner core. It is the movement of the fluid iron in the outer core that creates Earth’s magnetic field. The outer core is 2300 km thick, and has a density of 12 g/cm3.
The mantle extends from the outer core to just under Earth’s surface. It is 2900 km thick, and contains about 80% of the Earth’s volume. The mantle consists of iron and magnesium silicates and magnesium oxides, so it is more similar to the rocks of Earth’s surface than to the materials in the core. The mantle has a density of 4.5 g/cm3, and temperatures in the range of 1000-1500 oC. The uppermost layer of the mantle is more rigid, while the deeper regions are fluid, and it is the motion of fluid materials in the mantle that is responsible for plate tectonics. Magma that rises to the surface through volcanoes originates in the mantle.
The outermost layer is the crust, which forms the solid, rocky surface of the Earth. The crust averages 15-20 km thick, but in some places, such as under mountains, the crust can reach thicknesses of up to 100 km. There are two main types of crust; continental crust and oceanic crust that differ in a number of ways. Continental crust is thicker than oceanic crust, averaging 20-70 km thick, compared to 5-10 km for oceanic crust. Continental crust is also older than oceanic crust; the oldest rocks in continental crust are about 4.4 billion years old, while the oldest oceanic crust only goes back about 180 million years. Finally, the two types of crust differ in their composition. Continental crust is made largely of granite, while oceanic crust is mostly composed of basalt. Basalt contains a higher proportion of heavier elements like iron and magnesium, making oceanic crust denser than continental crust (3 g/cm3 vs. 2.7 g/cm3.) Granite also contains larger crystals than basalt, as underground or surface continental magmas can cool slowly, which allows time for crystal structures to form before the rocks solidify. Basalt also forms from cooling magmas, but exposure to air or water can cause them to cool faster, which does not allow time for large crystals to form.
Based on physical characteristics, we can also divide the outermost layers of Earth into the lithosphere and asthenosphere. The lithosphere consists of the crust and the cool, rigid, outer 80-100 km of the mantle. The crust and outer mantle moves together as a unit, so they are combined together into the lithosphere. The asthenosphere lies below the lithosphere, from about 100-200 km to about 670 km deep. It includes the more “plastic” softer region of the mantle, where fluid movements can occur. The solid lithosphere is thus floating on the fluid asthenosphere.
Isostasy
To help explain how the lithosphere is floating on the asthenosphere, we need to examine the concept of isostasy. Isostasy refers to the way a solid will float on a fluid. The relationship between the crust and the mantle is illustrated in Figure 1.5.2. On the right is an example of a non-isostatic relationship between a raft and solid concrete. It’s possible to load the raft up with lots of people, and it still won’t sink into the concrete. On the left, the relationship is an isostatic one between two different rafts and a swimming pool full of peanut butter. With only one person on board, the raft floats high in the peanut butter, but with three people, it sinks dangerously low. We’re using peanut butter here, rather than water, because its viscosity more closely represents the relationship between the crust and the mantle. Although it has about the same density as water, peanut butter is much more viscous (stiff), and so although the three-person raft will sink into the peanut butter, it will do so quite slowly.

The relationship of Earth’s crust to the mantle is similar to the relationship of the rafts to the peanut butter. The raft with one person on it floats comfortably high. Even with three people on it the raft is less dense than the peanut butter, so it floats, but it floats uncomfortably low for those three people. The crust, with an average density of around 2.6 grams per cubic centimeter (g/cm3), is less dense than the mantle (average density of approximately 3.4 g/cm3 near the surface, but more than that at depth), and so it is floating on the “plastic” mantle. When more weight is added to the crust, through the process of mountain building, it slowly sinks deeper into the mantle and the mantle material that was there is pushed aside (Figure 1.5.3, left). When that weight is removed by erosion over tens of millions of years, the crust rebounds and the mantle rock flows back (Figure 1.5.3, right).
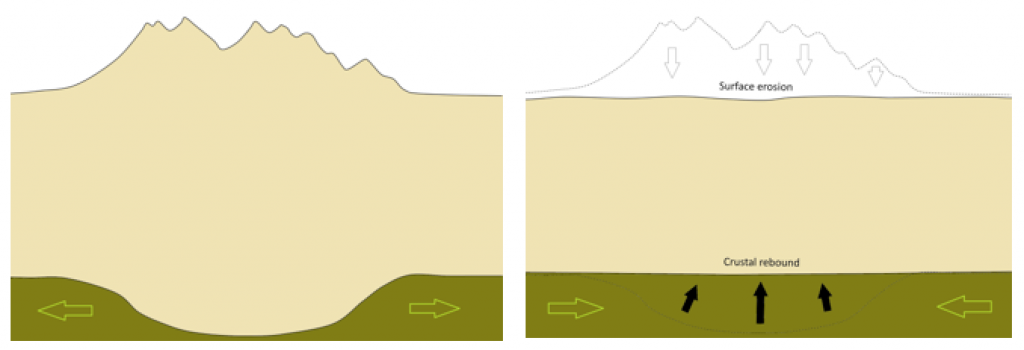
The crust and mantle respond in a similar way to glaciation. Thick accumulations of glacial ice add weight to the crust, and as the mantle beneath is squeezed to the sides, the crust subsides. When the ice eventually melts, the crust and mantle will slowly rebound, but full rebound will likely take more than 10,000 years. Large parts of Canada are still rebounding as a result of the loss of glacial ice over the past 12,000 years, and as shown in Figure 1.5.4, other parts of the world are also experiencing isostatic rebound. The highest rate of uplift is in within a large area to the west of Hudson Bay, which is where the Laurentide Ice Sheet was the thickest (over 3,000 m). Ice finally left this region around 8,000 years ago, and the crust is currently rebounding at a rate of nearly 2 cm/year.
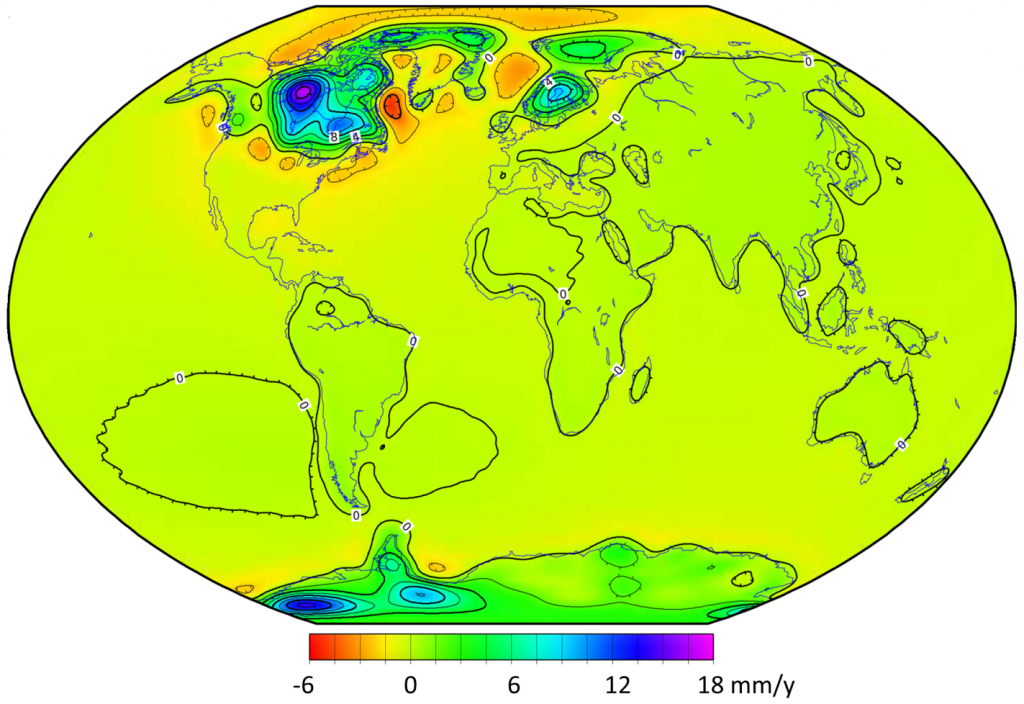
Since continental crust is thicker than oceanic crust, it will float higher and extend deeper into the mantle than oceanic crust. The boundary between the crust and the mantle is known as the Mohorovičić discontinuity (or Moho; see section 1.6). Crust is thickest where there are mountains, so the Moho will be deeper under mountains than under the oceanic crust. Since oceanic crust is also denser than continental crust, it floats lower on the mantle. Since the oceanic crust lies lower than the continental crust, and since water flows downhill to reach the lowest point, this explains why water has accumulated over the oceanic crust to form the oceans.
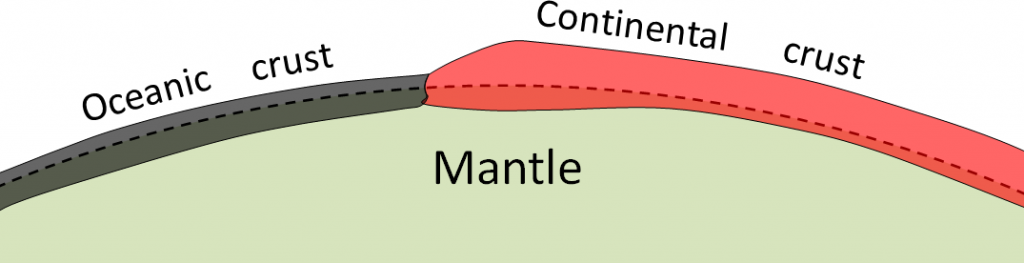
The balance of incoming and outgoing heat on Earth is referred to as its heat budget. As with any budget, to maintain constant conditions the budget must be balanced so that the incoming heat equals the outgoing heat. The heat budget of Earth appears below (Figure 6.1.1).
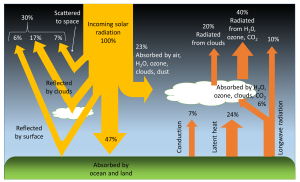
Of all of the solar energy reaching the Earth, about 30% is reflected back into space from the atmosphere, clouds, and surface of the Earth. Another 23% of the energy is absorbed by the water vapor, clouds, and dust in the atmosphere, where it is converted into heat. Just under half (47%) of the incoming solar radiation is absorbed by the land and ocean, and this energy heats up the Earth's surface. The energy absorbed by the Earth returns to the atmosphere through three processes; conduction, radiation, and latent heat (phase change)(Figure 6.1.1).
Conduction is the transfer of heat through direct contact between the surface and the atmosphere. Air is a relatively poor thermal conductor (which means it is a good insulator), so conduction represents only a small part of the energy transfer between the Earth and the atmosphere; equal to about 7% of the incoming solar energy.
All bodies with a temperature above absolute zero (-273o C) radiate heat in the form of longwave, infrared radiation (see the electromagnetic spectrum in section 5.9). The warmed Earth is no exception, and about 16% of the original solar energy is radiated from the Earth to the atmosphere (Figure 6.1.1). Some of this radiated energy will dissipate into space, but a significant amount of heat will be absorbed by the atmosphere. This is the basis for the greenhouse effect (Figure 6.1.2). In the greenhouse effect, shortwave solar radiation passes through the atmosphere and reaches the Earth's surface where it gets absorbed. When the radiation is re-emitted by the Earth, it is now in the form of long wavelength, infrared radiation, which does not easily pass through the atmosphere. Instead, this infrared radiation is absorbed by the atmosphere, particularly by the greenhouse gases such as CO2, methane, and water vapor. As a result, the atmosphere heats up. Without the greenhouse effect, the average temperature on Earth would be about -18o C, which is too cold for liquid water, and therefore life as we know it could not exist!

There is a great deal of concern about the greenhouse effect across the globe; not because of the presence of the effect itself, but because the effect is intensifying, causing climate change or global warming. Since the Industrial Revolution the atmospheric concentrations of the major greenhouse gases, particularly CO2 and methane, have increased dramatically due to industrialization, the burning of fossil fuels, and deforestation. At the same time, there has been rapid warming of the global climate; CO2 concentrations have increased more than 25% and global temperature has risen by 0.5o C over the past century. Unless production of these greenhouse gases is curbed, this rapid warming trend may continue, with potentially dire consequences. See section 6.5 for detailed information on the causes and effects of climate change.
The largest pathway for heat exchange between the land or oceans and the atmosphere is latent heat transferred through phase changes; heat released or absorbed when water moves between solid, liquid, and vapor forms (see section 5.1). Heat must be added to liquid water to make it evaporate, and when water vapor is formed, that heat is removed from the ocean and transferred to the atmosphere along with the water vapor. When water vapor condenses into rain, that heat is then returned to the oceans. The same process happens with the formation and melting of ice. Heat is absorbed by ice when it melts, and heat is released when ice forms, and these phase changes transfer heat between the oceans and the atmosphere.
To complete the heat budget, the heat that is absorbed by the atmosphere either directly from solar radiation or as a result of conduction, radiation and latent heat, is eventually radiated back into space (Figure 6.1.1).
Differential Heating of Earth's Surface
If the Earth was a flat surface facing the sun, every part of that surface would receive the same amount of incoming solar radiation. However, because the Earth is a sphere, sunlight is not equally distributed over the Earth's surface, so different regions of Earth will be heated to different degrees. This differential heating of Earth's surface occurs for a number of reasons. First, because of the curvature of Earth, sunlight only falls perpendicularly to the surface at the center of the sphere (equatorial regions). At any other point on Earth, the angle between the surface and the incoming solar radiation is less than 90o. Because of this, the same amount of incoming solar radiation will be concentrated in a smaller area at the equator, but will be spread over a much larger area at the poles (Figure 6.1.3). Thus the tropics receive more intense sunlight and a greater amount of heating per unit of area than the polar regions.

The angle at which sunlight strikes the Earth contributes to differential heating of the surface in an additional way. At the poles, because of the angle at which the solar energy strikes the surface, more of the light will glance off of the surface and the atmosphere and be reflected back into space. At the equator, the direct angle with which light reaches the surface results in more of the energy being absorbed rather than reflected. Finally, the poles reflect more solar energy than other parts of the Earth because the poles have a higher albedo. The albedo refers to reflectivity of a surface. Lighter surfaces are more reflective than darker surfaces (which absorb more energy), and therefore have a higher albedo. At the poles, the ice, snow and cloud cover create a much higher albedo, and the poles reflect more and absorb less solar energy than the lower latitudes. Through all of these mechanisms, the poles absorb much less solar radiation than equatorial regions, which is why the poles are cold and the tropics are very warm.
But there is an interesting twist to this global distribution of heat. The tropical regions actually receive more radiant heat than they emit, and the poles emit more heat than they receive (Figure 6.1.4). We should therefore expect that the tropics will be getting continually warmer, while the poles become increasingly cold. Yet this is not the case; so what is happening? Rather than the heat remaining isolated near the equator, about 20% of the heat from the tropics is transported to the poles before it is emitted. This large scale transport of energy moderates the climates at both extremes. The mechanisms for this heat transfer are ocean and atmospheric circulation, the topic of the next section.

The idea of differential heating of the Earth's surface is fundamental to understanding a wide range of oceanographic and atmospheric processes. This differential heating leads to atmospheric convection, which creates winds, which blow over the water and create waves and surface currents, and these currents influence nutrient distribution, which promotes primary production, which then supports the rest of the ocean ecosystem. So there's a lot riding on the simple fact that more light reaches the tropics than the poles!
The ocean is also home to a variety of birds. These birds are referred to as seabirds because they spend a considerable amount of time around the sea. Many seabirds spend their entire lives in the ocean, only coming to land to reproduce. Some seabirds dive and swim below the surface, such as penguins, whose wings are modified into flippers, or cormorants, whose webbed feet act as paddles. Others stay at the surface, only hunting in the uppermost meter of the water (Keddy). Please go to this website to read more about seabirds: https://ca.audubon.org/what-s-seabird
Birds evolved from reptilian ancestors
Birds split from the main reptile branch about 150 million years ago with an intermediate form known as Archaeopteryx, which was about the size of a crow. They have reptilian characteristics, such as their feet and lower legs are covered with scales and terminate in claws, their reproductive physiology is basically reptilian (laying eggs out of water), their feathers are believed to have been derived from scales, and have many other developmental and structural similarities with reptiles. Yet, unlike reptiles, birds are warm-blooded (endothermic) and maintain a constant internal body temperature.
Marine birds
Marine birds tend to be larger and stronger than their land counterparts. They have a lightweight, low-density body structure with hollow bones. Their wings are long, pointed and cupped underneath to support a large and muscular organism aloft for long periods of time.
They do not drink freshwater. Instead, they have salt glands over their eyes that remove the salt, thus permitting them to drink seawater, freeing them from dependence on freshwater from the land. The salty fluid is expelled through their bills.
The albatross
- This is one of the largest of the oceanic birds, with a wingspan of up to 12 feet
- They are the best gliders in the world – may remain aloft for months at a time, taking advantage of the west wind drift (winds that blow completely around Antarctica)
Pelicans
- This prehistoric-looking bird feeds by diving on its prey and entrapping it in its large gular pouch
- It has air sacs in its shoulders that absorb the impact with the water
Arctic Terns
- This bird feeds on small fish swimming near the surface that it catches by diving. It may even swim below the surface after them
- It lives at high latitudes and breeds on all far north rocky coasts
- However, it dislikes cold weather, so makes the longest migration of any animal, 15,000 miles each way
Penguins
- Penguins are found only in the southern hemisphere
- They are flightless birds that "fly" through the water after fish, squid and krill
- They slow their heart rate
- Have subcutaneous fat layers for insulation
- Have heavy plumage for insulation
- Their bodies are streamlined
- They have heavy feet for paddling and kicking against the water
- Their blood is shunted to the heart and brain and the extremities are deprived of blood, in order to slow heat loss
- These apply to most diving birds
Emperor Penguin: These are the largest diving birds in the world, up to 4 ft in height
- They're the best bird divers in the world, can routinely dive for 5-10 minutes (most bird dives last 30 seconds to a minute) and at best can stay down for 15-18 minutes, diving to over 1000 feet
- They eat small fish and krill – penguin populations have increased as a result of the killing off of the great whales – more krill is available as food for penguins
Penguin Adaptations
- They fluff their feathers to create trapped air in a dead space
- They have long feathers
- They rock back and forth on their heels on the ice to limit the area in contact with the ice and slow heat loss
- They can reduce blood flow to and heat loss from their wings and feet
- They exhibit "huddle" behavior – gathering in large groups and constantly changing position so that each gets a turn in the center, where it's warmest
The first paragraph, by Keddy (University of California, Davis), is shared under a not declared license and was authored, remixed, and/or curated by LibreTexts. Download this book for free at https://geo.libretexts.org/Courses/Diablo_Valley_College/OCEAN-101%3A_Fundamentals_of_Oceanography_(Keddy)
The rest was written by Dr. Cristina Cardona.
A whole new ecosystem reliant on the processes of plate tectonics was discovered on the deep seafloor of the Galapagos Rift in 1977. The deep sea submersible Alvin was exploring in 2500 m of water when it encountered unusually warm water. Following the temperature gradient, Alvin eventually discovered jets of superheated water coming from out of the seafloor at temperatures up to 350o C (the normal temperature for water at this depth would be 2-4o C). The water poured out of cracks in the crust, as well as through tall chimneys up to 20 m high and 1 m wide, and as it emerged it took on the appearance of thick black smoke, These fissures were named hydrothermal vents, and the chimneys "black smokers".
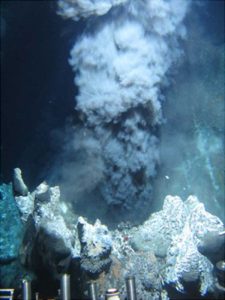
To create these vents, water percolates into the crust where there are plumes of magma close to the surface. The water gets superheated by the magma, then moves back to the surface through convection and is released through the vents. The hot water dissolves minerals from the surrounding rock, and as the water emerges and cools, the dissolved minerals and inorganic sulfides precipitate out as small particles and turn the water black, leading to the black "smoke" coming from the vents. Precipitation of these minerals also create the tall chimneys characteristic of many hydrothermal vents.
Since their original discovery in the Galapagos Rift, hydrothermal vents have been located across the globe along oceanic ridges where there is shallow crust and a lot of tectonic activity (Figure 2.11.2).
Figure 2.11.2 Distribution of hydrothermal vents (red dots) and their association with plate boundaries (By DeDuijn (Own work) [CC BY-SA 4.0], via Wikimedia Commons).
As unexpected as it was to discover these vent systems, even more surprising was the fact that they were teeming with life. The vents are surrounded by a diverse range of previously unknown organisms, including giant tube worms over 2 m long, crabs, shrimp, giant mussels, and mats of bacteria. How is it that such a diverse community can exist in the ocean depths, far removed from the sunlight that supports photosynthesis and primary production in most other ecosystems? The answer is that the water exiting the vents is rich in hydrogen sulfide (H2S), oxygen and CO2. The bacteria surrounding the vents use energy from the oxidation of sulfur compounds like H2S to form carbohydrates from CO2 and water. This is the process of chemosynthesis, and the bacteria are very productive as these reactions occur faster at high temperatures. The bacteria then represent the base of the food web, as other organisms eat the bacteria, or derive their energy from bacteria living symbiotically within their tissues. Watch the video below for more about hydrothermal vents.
https://www.youtube.com/watch?v=UVzBjY8oLkk
Our modern understanding of tide formation stems from Isaac Newton's Law of Universal Gravitation, which states that any two objects have a gravitational attraction to each other. The magnitude of the force is proportional to the masses of the objects, and inversely proportional to the square of the distance between the objects, according to the equation in Figure 3.5.1.
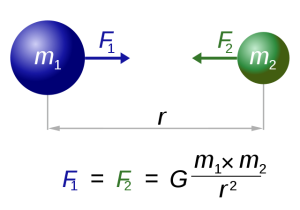
In the case of tides, there are a few other factors that modify this equation so that the distance (r) is cubed rather than squared, giving distance an even greater impact on tidal forces. But for our purposes, the important lesson is that the greater the masses of the objects, the greater the gravitational force, and the farther the objects are from each other, the weaker the force.
Such a gravitational force exists between the Earth and moon, attempting to pull them towards each other. Since the water covering Earth is fluid (unlike the solid land that is more resistant to tidal forces), this gravitational force pulls water towards the moon, creating a "bulge" of water on the side of the Earth facing the moon (Figure 3.5.2). This bulge always faces the moon, while the Earth rotates through it; the regions of Earth moving through the bulge experience a high tide, while those parts of the Earth away from the bulge experience a low tide.
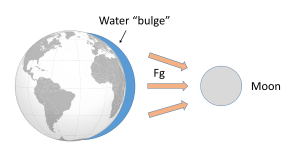
If the tides were this simple, everywhere on Earth would see one high tide per day, as there would only be a bulge of water on the side closest to the moon. However, if you have ever looked at tide charts, or lived near the ocean, you probably know that in most places there are two high tides and two low tides per day. Where is this second high tide "bulge" coming from?
The gravitational force between the Earth and moon might be expected to draw the two objects closer together, however, this is not happening. This is because the inward gravitational force is opposed by outward forces that keep the Earth and moon apart. The outward force is an intertial force created by the rotation of the Earth and moon. Contrary to popular belief, the moon is not simply rotating around the Earth; in fact, the Earth and moon are both rotating around each other. Imagine the Earth and moon as equal-sized objects revolving around a point at their center of mass. If both objects had the same mass, the center of rotation would be a point equidistant between the two objects. But since the mass of the Earth is 82 times greater than the mass of the moon, the center of revolution must be closer to the Earth. As an analogy, think about two people on a see-saw. If the people are of roughly equal size, they can sit on either end of the see-saw at it will rotate around a point at equal distance between them. But if the two people have very different masses, such as a large adult and a small child, the larger person must move closer to the pivot point for the see-saw to rotate effectively. In the same way, the center of rotation between the Earth and the moon (the barycenter) must be located closer to the Earth. In fact, the center of rotation lies within the Earth, about 1600 km below the surface. As the Earth and moon rotate around the barycenter, the moon travels much farther than the Earth, giving the impression that the moon is rotating around Earth (Figure 3.5.3).
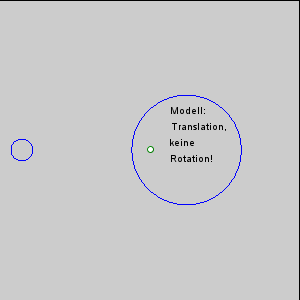
The rotation of the Earth-moon system creates an outward inertial force, which balances the gravitational force to keep the two bodies in their orbits. The inertial force has the same magnitude everywhere on Earth, and is always directed away from the moon. Gravitational force, on the other hand, is always directed towards the moon, and is stronger on the side of the Earth closest to the moon. Figure 3.5.4 describes how these forces combine to create the tidal forces. At point O in the center of the Earth, the gravitational force (Fg) and the inertial force (Fr) are equal, and cancel each other out. On the side of Earth closest to the moon, the inward gravitational force (Fg) is greater than the outward inertial force (Fr); the net resulting force (A) is directed towards the moon, and creates a bulge of water on the side facing the moon. On the side of Earth opposite the moon, the outward inertial force is greater than the inward gravitational force; the net resulting force (C) is directed away from the moon, creating a water bulge directed away from the moon.
Now, as the Earth rotates through a 24 hour day, each region passes through two bulges, and experiences two high tides and two low tides per day. This represents Newton's Equilibrium Theory of Tides, where there are two high tides and two low tides per day, of similar heights, each six hours apart. But as with everything else in oceanography, reality is much more complex than this idealized situation.
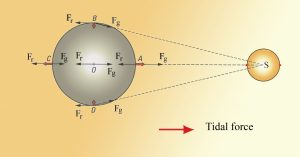
Some of the additional complexity is because in addition to the moon, the sun also exerts tide-affecting forces on Earth. The solar gravitational and inertial forces arise for the same reasons described above for the moon, but the magnitudes of the forces are different. The sun is 27 million times more massive than the moon, but it is 387 times farther away from the Earth. Despite its larger mass, because the sun is so much farther away than the moon, the sun's gravitational forces are only about half as strong as the moon's (remember that distance is cubed in the gravity equation). The sun thus creates its own, smaller water bulges, independent of the moon's, that contribute to the creation of tides.
When the sun, Earth and moon are aligned, as occurs during new and full moons, the solar and lunar bulges are also aligned, and add to each other (constructive interference; see section 4.2) creating an especially high tidal range; high high tides and low low tides (Figure 3.5.5). This period of maximum tidal range is called a spring tide, and they occur every two weeks.

When the sun, Earth and moon are at 90o to each other, the solar and lunar bulges are out of phase, and cancel each other out (destructive interference). Now the tidal range is small, with low high tides and high low tides (Figure 3.5.6). These are neap tides, and occur every two weeks, when the moon is in its 1/4 and 3/4 phases (Figure 3.5.7).


By Paul Webb, used under a CC-BY 4.0 international license. Download this book for free at https://rwu.pressbooks.pub/webboceanography/front-matter/preface/
Most of the waves discussed in the previous section referred to deep water waves in the open ocean. But what happens when these waves move towards shore and encounter shallow water? Remember that in deep water, a wave’s speed depends on its wavelength, but in shallow water wave speed depends on the depth (section 3.1). When waves approach the shore they will "touch bottom" at a depth equal to half of their wavelength; in other words, when the water depth equals the depth of the wave base (Figure 3.3.1). At this point their behavior will begin to be influenced by the bottom.
When the wave touches the bottom, friction causes the wave to slow down. As one wave slows down, the one behind it catches up to it, thus decreasing the wavelength. However, the wave still contains the same amount of energy, so while the wavelength decreases, the wave height increases. Eventually the wave height exceeds 1/7 of the wavelength, and the wave becomes unstable and forms a breaker. Often breakers will start to curl forwards as they break. This is because the bottom of the wave begins to slow down before the top of the wave, as it is the first part to encounter the seafloor. So the crest of the wave gets “ahead” of the rest of the wave, but has no water underneath it to support it (Figure 3.3.1).
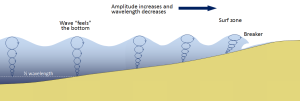
There are three main types of breakers: spilling, plunging, and surging. These are related to the steepness of the bottom, and how quickly the wave will slow down and its energy will get dissipated.
- Spilling breakers form on gently sloping or flatter beaches, where the energy of the wave is dissipated gradually. The wave slowly increases in height, then slowly collapses on itself (Figure 3.3.2). For surfers, these waves provide a longer ride, but they are less exciting.

- Plunging breakers form on more steeply-sloped shores, where there is a sudden slowing of the wave and the wave gets higher very quickly. The crest outruns the rest of the wave, curls forwards and breaks with a sudden loss of energy (Figure 3.3.3). These are the “pipeline” waves that surfers seek out.

- Surging breakers form on the steepest shorelines. The wave energy is compressed very suddenly right at the shoreline, and the wave breaks right onto the beach (Figure 3.3.4). These waves give too short (and potentially painful) a ride for surfers to enjoy.

Wave Refraction
Swell can be generated anywhere in the ocean and therefore can arrive at a beach from almost any direction. But if you have ever stood at the shore you have probably noticed that the waves usually approach the shore somewhat parallel to the coast. This is due to wave refraction. If a wave front approaches shore at an angle, the end of the wave front closest to shore will touch bottom before the rest of the wave. This will cause that shallower part of the wave to slow down first, while the rest of the wave that is still in deeper water will continue on at its regular speed. As more and more of the wave front encounters shallower water and slows down, the wave font refracts and the waves tend to align themselves nearly parallel to the shoreline (they are refracted towards the region of slower speed). As we will see in section 5.2, the fact that the waves do not arrive perfectly parallel to the beach causes longshore currents and longshore transport that run parallel to the shore.
Refraction can also explain why waves tend to be larger off of points and headlands, and smaller in bays. A wave front approaching shore will touch the bottom off of the point before it touches bottom in a bay. Once again, the shallower part of the wave front will slow down, and cause the rest of the wave front to refract towards the slower region (the point). Now all of the initial wave energy is concentrated in a relatively small area off of the point, creating large, high energy waves (Figure 3.3.6). In the bay, the refraction has caused the wave fronts to refract away from each other, dispersing the wave energy, and leading to calmer water and smaller waves. This makes the large waves of a “point break” ideal for surfing, while water is calmer in a bay, which is where people would launch a boat. This difference in wave energy also explains why there is net erosion on points, while sand and sediments get deposited in bays (see section 5.3).

By Paul Webb, used under a CC-BY 4.0 international license. Download this book for free at https://rwu.pressbooks.pub/webboceanography/front-matter/preface/
Written by Dr. Cristina Cardona.
In addition to fish, the ocean is also home to a variety of birds. These birds are referred to as seabirds because they spend a considerable amount of time around the sea. Many seabirds spend their entire lives in the ocean, only coming to land to reproduce. Some seabirds dive and swim below the surface, such as penguins, whose wings are modified into flippers, or cormorants, whose webbed feet act as paddles. Others stay at the surface, only hunting in the uppermost meter of the water (Keddy). Please go to this website to read more about seabirds: https://ca.audubon.org/what-s-seabird
Birds evolved from reptilian ancestors
Birds split from the main reptile branch about 150 million years ago with an intermediate form known as Archaeopteryx, which was about the size of a crow. They have reptilian characteristics, such as their feet and lower legs are covered with scales and terminate in claws, their reproductive physiology is basically reptilian (laying eggs), their feathers are believed to have been derived from scales, and have many other developmental and structural similarities with reptiles. Yet, unlike reptiles, birds are warm-blooded (endothermic) and maintain a constant internal body temperature.
Marine birds
Marine birds tend to be larger and stronger than their land counterparts. They have a lightweight, low-density body structure with hollow bones. Their wings are long, pointed and cupped underneath to support a large and muscular organism aloft for long periods of time.
They do not drink freshwater. Instead, they have salt glands over their eyes that remove the salt, thus permitting them to drink seawater, freeing them from dependence on freshwater from the land. The salty fluid is expelled through their bills.
The albatross
- This is one of the largest of the oceanic birds, with a wingspan of up to 12 feet
- They are the best gliders in the world – may remain aloft for months at a time, taking advantage of the west wind drift (winds that blow completely around Antarctica)
Pelicans
- This prehistoric-looking bird feeds by diving on its prey and entrapping it in its large gular pouch
- It has air sacs in its shoulders that absorb the impact with the water
Arctic Terns
- This bird feeds on small fish swimming near the surface that it catches by diving. It may even swim below the surface after them
- It lives at high latitudes and breeds on all far north rocky coasts
- However, it dislikes cold weather, so makes the longest migration of any animal, 15,000 miles each way
Penguins
- Penguins are found only in the southern hemisphere
- They are flightless birds that "fly" through the water after fish, squid and krill
- They slow their heart rate
- Have subcutaneous fat layers for insulation
- Have heavy plumage for insulation
- Their bodies are streamlined
- They have heavy feet for paddling and kicking against the water
- Their blood is shunted to the heart and brain and the extremities are deprived of blood, in order to slow heat loss
- These apply to most diving birds
Emperor Penguin: These are the largest diving birds in the world, up to 4 ft in height
- They're the best bird divers in the world, can routinely dive for 5-10 minutes (most bird dives last 30 seconds to a minute) and at best can stay down for 15-18 minutes, diving to over 1000 feet
- They eat small fish and krill – penguin populations have increased as a result of the killing off of the great whales – more krill is available as food for penguins
Penguin Adaptations
- They fluff their feathers to create trapped air in a dead space
- They have long feathers
- They rock back and forth on their heels on the ice to limit the area in contact with the ice and slow heat loss
- They can reduce blood flow to and heat loss from their wings and feet
- They exhibit "huddle" behavior – gathering in large groups and constantly changing position so that each gets a turn in the center, where it's warmest
The first paragraph, by Keddy (University of California, Davis), is shared under a not declared license and was authored, remixed, and/or curated by LibreTexts. Download this book for free at https://geo.libretexts.org/Courses/Diablo_Valley_College/OCEAN-101%3A_Fundamentals_of_Oceanography_(Keddy)
The rest was written by Dr. Cristina Cardona.
Protecting the Marine Environment
Many national, state, and local governments and organizations have been struggling for decades to manage marine pollution and protect coastal environments. In the United States, the National Oceanographic and Atmospheric Administration (NOAA) overseas large portions of the coastal waters around North America, attempting to stop overfishing and restricting coastal development in many regions. Expanding efforts involve protecting coastal wetlands, mitigating coastal hazards, ensuring public coastal access, protecting beaches and coastal park lands, locating of energy and government facilities, and managing sensitive habitats, fishery areas, and aquaculture. Efforts are underway nationwide to prevent and control polluted runoff by replacing outdated storm water runoff and sewage systems, reducing agricultural pollutants, preventing or mitigating development within sensitive habitats and erosion-prone areas, and finding ways for communities to reduce refuse and debris from entering coastal waters.
Focus on Coral Reefs
Coral reefs (or coral ecosystems) are among the most important and also most sensitive habitats throughout the world’s oceans. Reefs provide habitat, spawning and nursery grounds for economically important fish species, and are hotspots of marine biodiversity. For humanity, coral reefs provide billions of dollars in economic and environmental benefits, including fishing, coastal protection, recreation, and tourism. Hundreds of millions of people worldwide depend of reef ecosystems for their livelihoods and food. However, coral ecosystems face serious threats from unsustainable fishing and land-based pollution (Figures 17-30 and 17-31).
Unfortunately, many of the world’s reefs have already been destroyed or severely damaged by pollution, unsustainable fishing practices, disease, introduction of invasive species, ship groundings, uncontrolled coastal development and other impacts.
Human activities are a primary cause for reef destruction. Pollutants from expanding coastal communities find their way to shallow coastal waters dominated by coral reefs, mostly in warm tropical waters. Many of the sea creatures, particularly invertebrates that attach to the seabed and filter seawater. Tourist visiting reefs step on fragile reef structures, introduce chemicals (such as zinc and other compounds in sun screen). Sewage and urban runoff carries silt (increasing turbidity) and introduce toxins that impact or kill reef organisms. Perhaps most alarming are the impacts of changes in water temperature and water chemistry associated with climate change.
Climate Change - The most important environmental issue of our times!
Studies conducted throughout the world’s oceans show the coral ecosystems are showing the detrimental effects of climate change caused by the burning of fossil fuels, deforestation, and bad agricultural practices that release large quantities of greenhouse gases into the atmosphere.
Climate change impacts coral ecosystems by increasing sea-surface temperatures and increased carbon dioxide levels in seawater. Long-term studies of CO2 concentrations in seawater show trends in reducing calcification rates in reef-building and reef-associated organisms. Increased sea surface temperature leads to coral bleaching (a result in the loss of symbiotic algae and bacteria) and death of skeletal reef-building organism. Weakened coral communities are susceptible to infection disease. Pollution from coastal development and agricultural runoff can also impede coral growth and reproduction, disrupt ecological functions, and cause disease.
Coal is the most carbon-intensive fossil fuel, producing the most carbon dioxide per unit volume burned. For every ton of coal burned, approximately 2.5 tons of CO2 is released into the air. Globally, coal is the largest-used fossil fuel source and the highest production of carbon dioxide emissions. Although coal represents only about one-third of share of fossil fuels consumed by the world’s total primary energy supply, coal is responsible for 43% of carbon dioxide emissions from burning fossil fuels.
Politics of Climate Change: Sadly, our world may be in big trouble because of the economics associated with energy and agricultural demands of a growing world population. Failure to address carbon emissions and the resultant impacts of rising temperatures and ocean acidification could make many marine and coastal management efforts futile. While reducing CO2 and other greenhouse gas emissions is vital to stabilize the global climate is essential, the excess that already exists in the atmosphere will persist throughout the next century.
Climate changes will have many impacts on marine systems including reduction in marine biodiversity, sea level is rising, and long-term forecasts predict changes in the frequency, intensity, and distribution of tropical storms as atmospheric and ocean circulation patterns change.
Politics vs. Technology: The key to saving or destroying our natural environments
The climate change issues will be every-increasingly important as the impacts become increasingly obvious as the number of natural and man-made disasters steadily rises. Humans have to collectively choose, through political means, to make the choices to change to cleaner technologies, and protecting and managing resources. The choices to move away from fossil-fuel consumption to alternative energy sources will be expensive and a hard fight because it will impact the livelihoods of many people (such as coal miners and workers in the petroleum industries). Predicted sea-level rise and global warming will impact all world's communities, both human and ecosystems. There will be many winners and losers in the transition to a cleaner, more sustainable world. Accepting the consequences for climate change will create many new jobs in the process. The longer humanity waits to make these changes, the greater the environmental problems will be in the future. "We can't fool mother nature!"
From Miracosta College, is shared under a not declared license and was authored, remixed, and/or curated by LibreTexts. Download this book for free at https://geo.libretexts.org/Bookshelves/Oceanography/Oceanography_101_(Miracosta)
Large marine disasters involving petroleum spills have happened many times over the past century. The impact of these events depends on where and how they occur. Some are accidents, others include intentional acts of war, such as the destruction of Kuwait’s oil fields in the First Gulf War. Oil spills can have a wide variety of impacts ranging from minimal (when far from coastal regions) to catastrophic when they impact shore regions. Two of the largest (most expensive) petroleum-related disasters affecting North American coastal waters are discussed below.
The Deepwater Horizon Disaster, 2010
The Deepwater Horizon Disaster started on April 20, 2010 with an explosion and fire of a submersible drilling platform located in the Gulf of Mexico about 40 miles (64 km) offshore from the Louisiana coast. The drilling operation involved tapping an oil reservoir deep in sedimentary deposits on the offshore region beyond the continental shelf. Problems with the drilling operation and failure of equipment to prevent a blowout that resulted in the explosion and fire, and the eventual sinking of the drilling platform two days later. 17 platform workers were killed and another 11 were injured by the explosion and fire. The open well, sheared of at the seabed, proceeded to spew large quantities of crude oil into Gulf waters until it was shut down in mid July when a 75 ton cap was put in place, sealing off the well. The disaster resulted in the largest oil spill in the history of the Petroleum Industry.
An estimated 200 million gallons (about 5 million barrels) of oil poured into ocean from the unconstrained well. Some of oil stayed on or near the seabed, much of it formed a large plum in layers within the ocean waters, and some migrated to the surface where wind and currents dispersed it. An extensive cleanup effort was undertaken to trap, degrade and disperse, or burn off much of the oil on the surface. Unfortunately, large amounts found its way onshore, impacting beaches and coastal wetlands, and severely impacting wildlife. The bad publicity wreaked economic havoc on coastal communities and businesses involved in fishing and recreation from Texas to Florida. As of 2015, BP (the company that operated the drilling program) agreed to pay $18.7 billion to settle all federal and state claims for the disaster - the biggest pollution penalty in U.S. history. Total settlement costs was in the range of $54 billion.
Settlement of all federal and state claims brings total costs to nearly $54 billion. BP PLC agreed to pay $18.7 billion to settle all federal and state claims arising from the 2010 Deepwater Horizon oil spill, including the biggest pollution penalty in U.S. history.
Because the spill happened in the warm open ocean waters, much of the crude oil from the spill eventually dispersed (evaporated or diluted) or was consumed by microbial activity.
![]() |
||
Figure 12.10.2. An oil slick spreads on the Gulf of Mexico from the Deep Horizon disaster. |
Exxon Valdez Oil Spill Disaster, 1989
Prior to the Deepwater Horizon disaster, the worst petroleum-related disaster affecting the US coastline was the Exxon Valdez oil spill (Figures 17-24 and 17-25). The oil spill occurred in Prince William Sound in the Gulf of Alaska. The Exxon Valdez, a large oil tanker bound for refineries in Long Beach, California, veered off course and struck a submerged rock “reef” outcrop on March 24, 1989. The disaster was blamed on poor navigation by a drunken ship captain, poorly trained personnel, and faulty and unused navigation equipment.
Estimates by governmental and other sources suggest that at least 10 to 11 million gallons (about 250,000 barrels) of Alaskan crude oil spilled into the coastal waters. The spill, so close to shore, eventually impaction about 1,300 miles (2,000 km) of coastline in the Gulf of Alaska (closer to 9,000 miles [14,500 km] considering all the islands, headlands, and bays along the rugged coastline). Rough seas, and the rugged and remote coastline made clean-up efforts extremely difficult, and the cold-water setting hampered the rapid decay and dispersion of the oil. The spill devastated habitats for salmon, seals, seabirds, and sea otters, and had a catastrophic effect of coastal communities in the region. The cost of the disaster, spread over many years, was in the range of about $7 billion. Hard facts were learned from the disaster. It turns out that some of the beach areas were "cleaned" - basically cooked with 150 F water. These areas were actually harmed more by the cleaning processes used. It was determined that about 35% of the oil evaporated, 8% burned, 5% dispersed by surf , and only about 5% biodegraded; the rest formed slicks that dispersed into the greater ocean currents offshore.
An outcome of the disaster is that all new large petroleum-transport vessels are now being built with double hulls to hopefully prevent future transport-spill disasters.
Impact of Petroleum Pollution on Wildlife
Pollution from petroleum-source products is a major problem in parts of the worlds oceans and coastlines. In addition, tar-ball, waxes, and other petroleum-derivative products can now be found throughout the world’s oceans. Evidence of the pollution is most abundant along developed (urban and industrial) coastlines where accidental spills occur most frequently. The risks of spills occur along all infrastructure systems associated with the petroleum production, refining, transportation, and consumption. Leaky oil from cars and trucks are a major non-point source of water pollution. Large oil spills and oil production disasters are some of the most costly, devastating both wildlife and the economic livelihoods of communities in regions where they occur (Figure 17.26).
Oil spills are particularly bad for homeothermic (warm blooded) organisms with fur of feathers. Saturation with oil causes these animals to loose insulation and they die from hypothermia. Oil slicks poison kill 150-450,000 sea birds killed each year. Organisms living in the intertidal zone most sensitive to oil contamination.
Refined oil and oil-derivative products tend to be non-biodegradable, and are more toxic to wildlife.
From Miracosta College, is shared under a not declared license and was authored, remixed, and/or curated by LibreTexts. Download this book for free at https://geo.libretexts.org/Bookshelves/Oceanography/Oceanography_101_(Miracosta)
Waves generally begin as a disturbance of some kind, and the energy of that disturbance gets propagated in the form of waves. We are most familiar with the kind of waves that break on shore, or rock a boat at sea, but there are many other types of waves that are important to oceanography:
- Internal waves form at the boundaries of water masses of different densities (i.e. at a pycnocline), and propagate at depth. These generally move more slowly than surface waves, and can be much larger, with heights exceeding 100 m. However, the height of the deep wave would be unnoticeable at the surface.
- Tidal waves are due to the movement of the tides. What we think of as tides are basically enormously long waves with a wavelength that may span half the globe (see section 4.1). Tidal waves are not related to tsunamis, so don’t confuse the two.
- Tsunamis are large waves created as a result of earthquakes or other seismic disturbances. They are also called seismic sea waves (section 3.4).
- Splash waves are formed when something falls into the ocean and creates a splash. The giant wave in Lituya Bay that was described in the introduction to this chapter was a splash wave.
- Atmospheric waves form in the sky at the boundary between air masses of different densities. These often create ripple effects in the clouds (Figure 3.1.1).
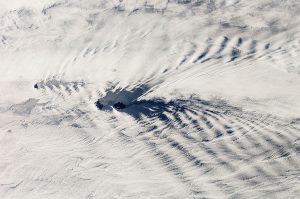
There are several components to a basic wave (Figure 3.1.2):
- Still water level: where the water surface would be if there were no waves present and the sea was completely calm.
- Crest: the highest point of the wave.
- Trough: the lowest point of the wave.
- Wave height: the distance between the crest and the trough.
- Wavelength: the distance between two identical points on successive waves, for example crest to crest, or trough to trough.
- Wave steepness: the ratio of wave height to length (H/L). If this ratio exceeds 1/7 (i.e. height exceeds 1/7 of the wavelength) the wave gets too steep, and will break.
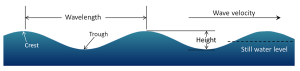
There are also a number of terms used to describe wave motion:
- Period: the time it takes for two successive crests to pass a given point.
- Frequency: the number of waves passing a point in a given amount of time, usually expressed as waves per second. This is the inverse of the period.
- Speed: how fast the wave travels, or the distance traveled per unit of time. This is also called celerity (c), where
c = wavelength x frequency
Therefore, the longer the wavelength, the faster the wave.
Although waves can travel over great distances, the water itself shows little horizontal movement; it is the energy of the wave that is being transmitted, not the water. Instead, the water particles move in circular orbits, with the size of the orbit equal to the wave height (Figure 3.1.3). This orbital motion occurs because water waves contain components of both longitudinal (side to side) and transverse (up and down) waves, leading to circular motion. As a wave passes, water moves forwards and up over the wave crests, then down and backwards into the troughs, so there is little horizontal movement. This is evident if you have ever watched an object such as a seabird floating at the surface. The bird bobs up and down as the wave pass underneath it; it does not get carried horizontally by a single wave crest.
Figure 3.1.3 Animation showing the orbital motion of particles in a surface wave (By Kraaiennest (Own work) [GFDL (http://www.gnu.org/copyleft/fdl.html) or CC BY-SA 4.0], via Wikimedia Commons).
The circular orbital motion declines with depth as the wave has less impact on deeper water and the diameter of the circles is reduced. Eventually at some depth there is no more circular movement and the water is unaffected by surface wave action. This depth is the wave base and is equivalent to half of the wavelength (Figure 3.1.4). Since most ocean waves have wavelengths of less than a few hundred meters, most of the deeper ocean is unaffected by surface waves, so even in the strongest storms marine life or submarines can avoid heavy waves by submerging below the wave base.
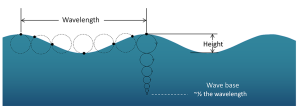
When the water below a wave is deeper than the wave base (deeper than half of the wavelength), those waves are called deep water waves. Most open ocean waves are deep water waves. Since the water is deeper than the wave base, deep water waves experience no interference from the bottom, so their speed only depends on the wavelength:
[latex]\text{speed (m/s)} = \sqrt{\frac{gL}{2\pi}}[/latex]
where g is gravity and L is wavelength in meters. Since g and π are constants, this can be simplified to:
[latex]\text{speed (m/s)} = 1.25\sqrt{L}[/latex]
Shallow water waves occur when the depth is less than 1/20 of the wavelength. In these cases, the wave is said to "touch bottom" because the depth is shallower than the wave base so the orbital motion is affected by the seafloor. Due to the shallow depth, the orbits are flattened, and eventually the water movement becomes horizontal rather than circular just above the bottom. The speed of shallow water waves depends only on the depth:
[latex]\text{speed (m/s)} = \sqrt{gd}[/latex]
where g is gravity and d is depth in meters. This can be simplified to:
[latex]\text{speed (m/s)} = 3.13\sqrt{d}[/latex]
Intermediate or transitional waves are found in depths between ½ and 1/20 of the wavelength. Their behavior is a bit more complex, as their speed is influenced by both wavelength and depth. The speed of an intermediate wave is calculated as:
which contains both depth and wavelength variables.