4 1.4 Mapping the Seafloor
The previous sections included some information on the depths of the oceans in various places. So how are we able to map the ocean floor to ascertain these vast depths?
To map the ocean floor we need to know the depth at a number of places. The process of measuring the depths is known as bathymetry. These measurements were first made through soundings, where a weighted line (lead line) was let out by hand until it touched the bottom, and the depth could be recorded from the length of the line (Figure 1.4.1). This technique led to the fathom as a unit of depth; as sailors hauled in the sounding line they would stretch it out to cover their arm span. The average arm span of a sailor was about six feet, so one fathom equals six feet, and the sailors could simply count the number of “arm spans” as they pulled in the line.
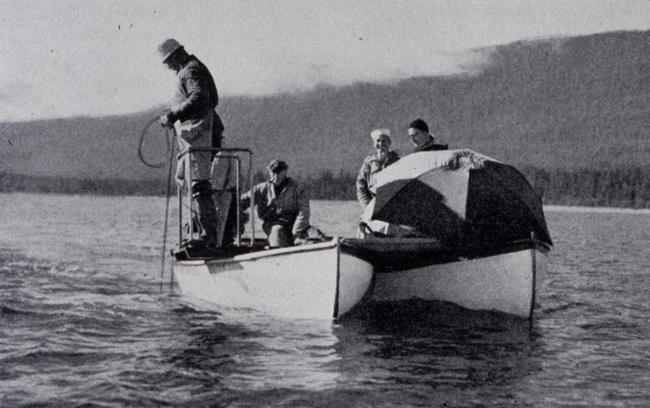
This technique had a number of drawbacks, and was usually limited to shallower water. It was very time consuming, and only gave depth data for a single point, so many individual soundings were needed to map an area. It could also be error-prone; in deep water it could be difficult to determine when the weight hit the bottom as the weight of the line itself could cause the line to keep sinking, and currents could deflect the line away from vertical, thus overestimating the depth. In later years, winches and heavy steel cables were used for deeper water, but this did not solve all of the problems inherent in the sounding method, and also added the constraint of excessive weight of the equipment.
In the 19th century, a number of modifications were made to this simple design. In 1802 the British clockmaker Edward Massey invented a mechanical device that was attached to the sounding line; as the device sank, a rotor turned a dial which locked in place when the line hit bottom (Figure 1.4.2). The line could then be reeled in and the depth read from the dial. In 1853 American sailor John Mercer Brooke developed a cannonball weight attached to a twine reel. The cannonball was dropped over the side and allowed to free-fall to the bottom; by timing the fall rate (the rate at which the twine unspooled) and noting when the rate changed as the cannonball hit the bottom, the water depth could be calculated. When it hit bottom, the cannonball was released and the line could be hauled back in, bringing with it a sample of mud in the iron bar that held the cannonball, thus confirming that the bottom had been reached.
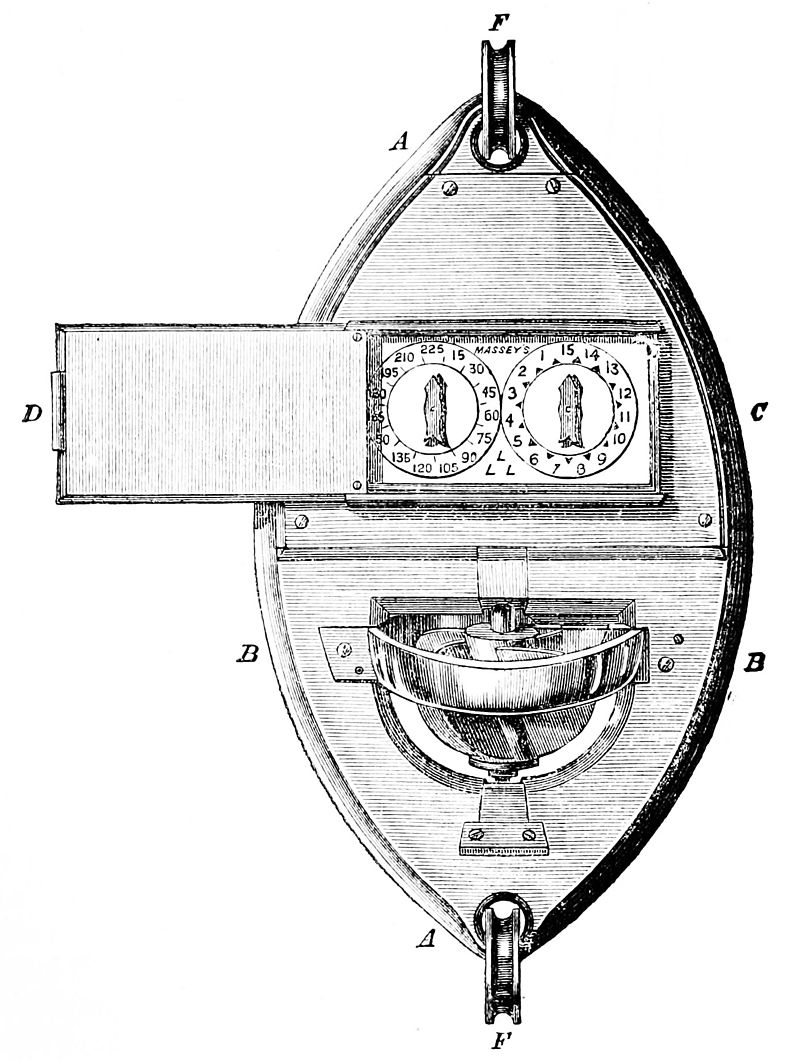
After the Titanic disaster in 1912, there was an effort to develop better methods of detecting icebergs from a ship. This led to the development of sonar (SOund Navigation And Ranging) technology, which was soon applied to mapping bathymetry. A sonar device called an echosounder sends out a pulse of sound, then listens for the returning echo. The timing of the returning echo is used to calculate depth. We know that the speed of sound in water is approximately 1500 m/s (see section 6.4). Since the returning echo traveled to the bottom and back, the water depth corresponds to half the time it takes for an echo to return, multiplied by the speed of sound in water (Figure 1.4.3):
depth=1/2*(two-way travel time)*(speed of sound in water)
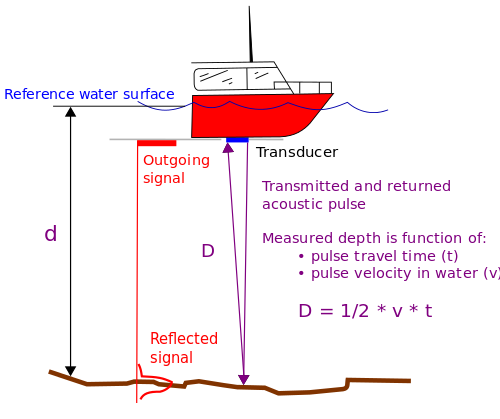
Echosounders allowed a fast, continuous record of bathymetry under a moving ship. However, they only give the depth directly under a ship’s path. Today, high resolution seafloor maps are made through multibeam or side scan sonar, either from a ship or from a towed transmitter (Fig. 1.4.4). Multibeam sonar produces a fan-shaped acoustic field allowing a much a wider area (>10 km wide) to be mapped simultaneously.
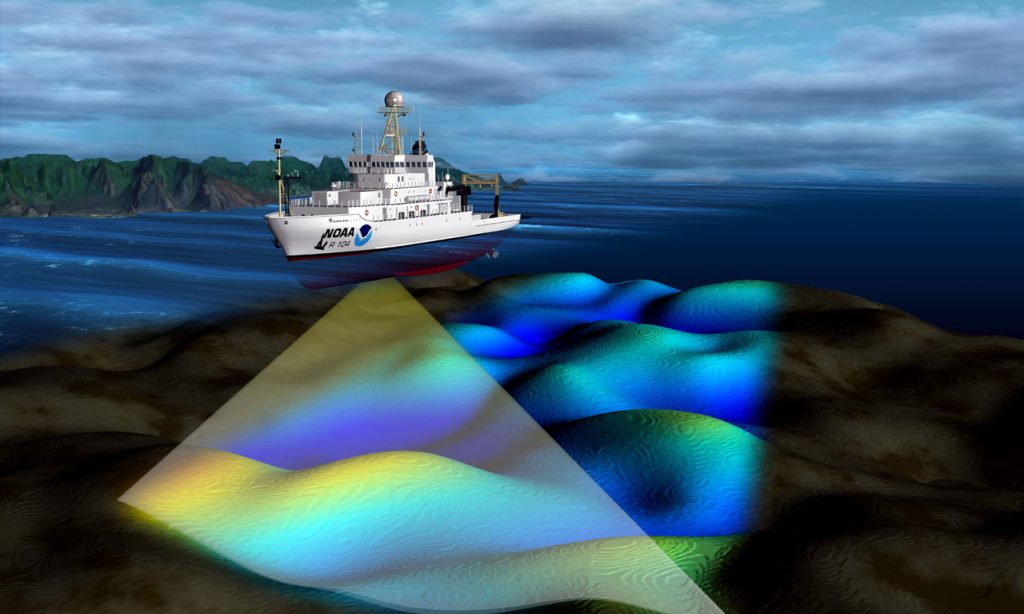
Large-scale mapping of the ocean floor is also carried out by satellites (originally SEASAT, then GEOSAT, now the Jason satellites) which use radio waves to measure the height of the sea surface (radar altimetry). The sea surface is not flat; gravity causes it to be slightly higher over elevated features on the ocean floor, and slightly lower over trenches and other depressions. Satellites send out radio waves, and similar to an echosounder, can use the returning waves to detect differences in sea surface height down to 3-6 cm (Figure 1.4.5). These differences in sea surface heights allow us to determine the topography under the surface. Unlike the old lead line technology, where hundreds of soundings were necessary to map a small area, each taking an hour or more to complete, the current satellites can map over 90% of Earth’s ice-free sea surface every 10 days!
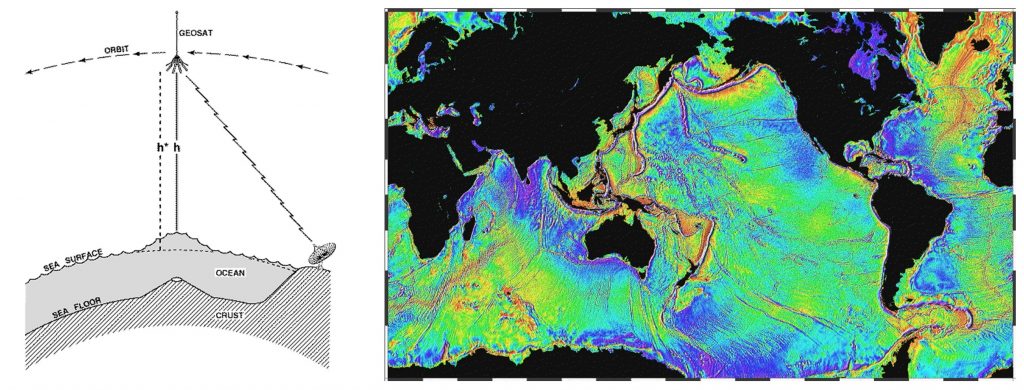
Scientist Spotlight: Marie Tharp
Watch this short video to learn how Marie Tharp became a pioneer in mapping the seafloor and provided important evidence for the theory of plate tectonics.
Additional links for more information:
- NOAA page on side scan sonar: https://oceanexplorer.noaa.gov/technology/sonar/side-scan.html
- NOAA page on exploring the ocean using satellite altimetry data: https://oceanservice.noaa.gov/facts/satellites-ocean.html
- NASA animations showing how the land masses of Earth would change if the oceans were drained: https://svs.gsfc.nasa.gov/4823
- By Paul Webb, used under a CC-BY 4.0 international license. Download this book for free at https://rwu.pressbooks.pub/webboceanography/front-matter/preface/
The most obvious feature of the oceans is that they contain water. Water is so ubiquitous that it may not seem like a very interesting substance, but it has many unique properties that impact global oceanographic and climatological processes. Many of these processes are due to hydrogen bonds forming between water molecules.
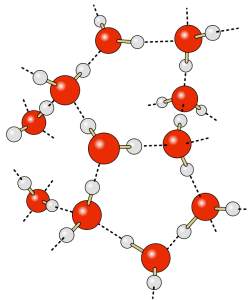
The water molecule consists of two hydrogen atoms and one oxygen atom. The electrons responsible for the bonds between the atoms are not distributed equally throughout the molecule, so that the hydrogen ends of water molecules have a slight positive charge, and the oxygen end has a slight negative charge, making water a polar molecule. The negative oxygen side of the molecule forms an attraction to the positive hydrogen end of a neighboring molecule. This rather weak force of attraction is called a hydrogen bond (Figure 5.1.1). If not for hydrogen bonds, water would vaporize at -68o C, meaning liquid water (and thus life) could not exist on Earth. These hydrogen bonds are responsible for some of water’s unique properties:
1. Water is the only substance to naturally exist in a solid, liquid, and gaseous form under the normal range of temperatures and pressures found on Earth. This is due to water’s relatively high freezing and vaporizing points (see below).
2. Water has a high heat capacity, which is the amount of heat that must be added to raise its temperature. Specific heat is the heat required to raise the temperature of 1 g of a substance by 1o C. Water has the highest specific heat of any liquid except ammonia (Table 5.1.1).
Table 5.1.1 Specific heat values for a number of common substances
Specific Heat (calories/g/Co) | |
---|---|
Ammonia | 1.13 |
Water | 1.00 |
Acetone | 0.51 |
Grain Alcohol | 0.23 |
Aluminum | 0.22 |
Copper | 0.09 |
Silver | 0.06 |
Water is therefore one of the most difficult liquids to heat or cool; it can absorb large amounts of heat without increasing its temperature. Remember that temperature reflects the average kinetic energy of the molecules within a substance; the more vigorous the motion, the higher the temperature. In water, the molecules are held together by hydrogen bonds, and these bonds must be overcome to allow the molecules to move freely. When heat is added to water the energy must first go to breaking the hydrogen bonds before the temperature can begin to rise. Therefore, much of the added heat is absorbed by breaking H bonds, not by increasing the temperature, giving water a high heat capacity.
Hydrogen bonds also give water a high latent heat; the heat required to undergo a phase change from solid to liquid, or liquid to gas. The latent heat of fusion is the heat required to go from solid to liquid; 80 cal/g in the case of ice melting to water. Ice is a solid because hydrogen bonds hold the water molecules into a solid crystal lattice (see below). As ice is heated, the temperature rises up to 0o C. At that point, any additional heat goes to melting the ice by breaking the hydrogen bonds, not to increasing the temperature. So as long as ice is present, the water temperature will not increase. This is why your drink will remain cold as long as it contains ice; any heat absorbed goes to melting the ice, not to warming the drink.
When all of the ice is melted, additional heat will increase the temperature of the water 1o C for each calorie of heat added, until it reaches 100o C. At that point, any additional heat goes to overcoming the hydrogen bonds and turning the liquid water into water vapor, rather than increasing the water temperature. The heat required to evaporate liquid water into water vapor is the latent heat of vaporization which has a value of 540 cal/g (Figure 5.1.2).
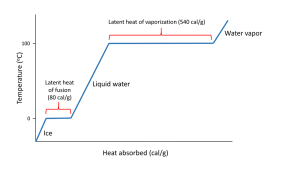
The high heat capacity of water helps regulate global climate, as the oceans slowly absorb and release heat, preventing rapid swings in temperature (see section 8.1). It also means that aquatic organisms aren't as subjected to the same rapid temperature changes as terrestrial organisms. A deep ocean organism may not experience more than a 0.5o C change in temperature over its entire life, while a terrestrial species may encounter changes of more than 20o C in a single day!
3. Water dissolves more substances than any other liquid; it is a "universal solvent", which is why so many substances are dissolved in the ocean. Water is especially good at dissolving ionic salts; molecules made from oppositely charged ions such as NaCl (Na+ and Cl-). In water, the charged ions attract the polar water molecules. The ions get surrounded by a layer of water molecules, weakening the bond between the ions by up to 80 times. With the bonds weakened between ions, the substance dissolves (Figure 5.1.3).
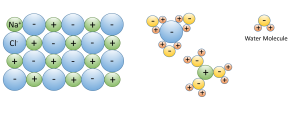
4. The solid phase is less dense than the liquid phase. In other words, ice floats. Most substances are denser in the solid form than in the liquid form, as their molecules are more closely packed together as a solid. Water is an exception: the density of fresh water is 1.0 g/cm3, while the density of ice is 0.92 g/cm3, and once again, this is due to the action of hydrogen bonds.
As water temperature cools the molecules slow down, eventually slowing enough that hydrogen bonds can form and hold the water molecules in a crystal lattice. The molecules in the lattice are spaced farther apart than the molecules in liquid water, which makes ice less dense than liquid water (Figure 5.1.4). This is familiar to anyone who has ever left a full water bottle in the freezer, only to have it burst as the water freezes and expands.
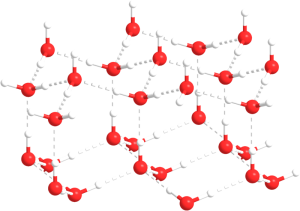
But the relationship between temperature and water density is not a simple linear one. As water cools, its density increases as expected, as the water molecules slow down and get closer together. However, fresh water reaches its maximum density at a temperature of 4o C, and as it cools beyond that point its density declines as the hydrogen bonds begin to form and the intermolecular spacing increases (Figure 5.1.5 inset). The density continues to decline until the temperature reaches 0o C and ice crystals form, reducing the density dramatically (Figure 5.1.5).
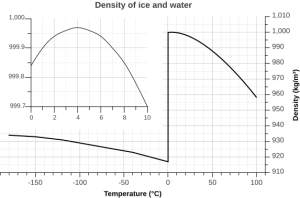
There are a number of important implications to ice being less dense than water. Ice floating on the surface of the ocean helps regulate ocean temperatures, and therefore global climate, by influencing the amount of sunlight that is reflected rather than absorbed (see section 5.6). On a smaller scale, surface ice can prevent lakes and ponds from freezing solid during the winter. As fresh surface water cools, the water gets denser, and sinks to the bottom. The new surface water then cools and sinks, and the process is repeated in what is referred to as overturning, with denser water sinking and less dense water moving to the surface only to be cooled and sink itself. In this way, the entire body of water is cooled somewhat evenly. This process continues until the surface water cools below 4o C. Below 4o C, the water becomes less dense as it cools, so it no longer sinks. Instead, it remains as the surface, getting colder and less dense, until it freezes at 0o C. Once fresh water freezes, the ice floats and insulates the rest of the water beneath it, reducing further cooling. The densest bottom water is still at 4o C, so it does not freeze, allowing the bottom of a lake or pond to remain unfrozen (which is good news for the animals living there) no matter how cold it gets outside.
The dissolved salts in seawater inhibit the formation of the crystal lattice, and therefore make it harder for ice to form. So seawater has a freezing point of about -2o C (depending on salinity), and freezes before a temperature of maximum density is reached. Thus seawater will continue to sink as it gets colder, until it finally freezes.
5. Water has a very high surface tension, the highest of any liquid except mercury (Table 5.1.2). Water molecules are attracted to each other by hydrogen bonds. For molecules not at the water surface, they are surrounded by other water molecules in all directions, so the attractive forces are evenly distributed in all directions. But for molecules at the surface there are few adjacent molecules above them, only below, so all of the attractive forces are directed inwards, away from the surface (Figure 5.1.6). This inwards force is what causes water droplets to take on a spherical shape, and water to bead up on a surface, as the spherical shape provides the minimum possible surface area. These attractive forces also cause the surface of the water to act like an elastic "skin" which allows things like insects to sit on the water's surface without sinking.
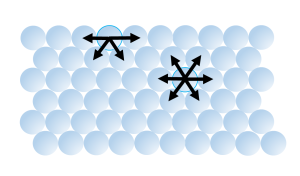
Table 5.1.2 Surface tensions of various liquids
Liquid | Surface Tension (millinewton/meter) | Temperature oC |
---|---|---|
Mercury | 487.00 | 15 |
Water | 71.97 | 25 |
Glycerol | 63.00 | 20 |
Acetone | 23.70 | 20 |
Ethanol | 22.27 | 20 |
By Paul Webb, used under a CC-BY 4.0 international license. Download this book for free at https://rwu.pressbooks.pub/webboceanography/front-matter/preface/
The most obvious feature of the oceans is that they contain water. Water is so ubiquitous that it may not seem like a very interesting substance, but it has many unique properties that impact global oceanographic and climatological processes. Many of these processes are due to hydrogen bonds forming between water molecules.
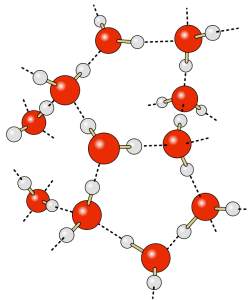
The water molecule consists of two hydrogen atoms and one oxygen atom. The electrons responsible for the bonds between the atoms are not distributed equally throughout the molecule, so that the hydrogen ends of water molecules have a slight positive charge, and the oxygen end has a slight negative charge, making water a polar molecule. The negative oxygen side of the molecule forms an attraction to the positive hydrogen end of a neighboring molecule. This rather weak force of attraction is called a hydrogen bond (Figure 5.1.1). If not for hydrogen bonds, water would vaporize at -68o C, meaning liquid water (and thus life) could not exist on Earth. These hydrogen bonds are responsible for some of water’s unique properties:
1. Water is the only substance to naturally exist in a solid, liquid, and gaseous form under the normal range of temperatures and pressures found on Earth. This is due to water’s relatively high freezing and vaporizing points (see below).
2. Water has a high heat capacity, which is the amount of heat that must be added to raise its temperature. Specific heat is the heat required to raise the temperature of 1 g of a substance by 1o C. Water has the highest specific heat of any liquid except ammonia (Table 5.1.1).
Table 5.1.1 Specific heat values for a number of common substances
Specific Heat (calories/g/Co) | |
---|---|
Ammonia | 1.13 |
Water | 1.00 |
Acetone | 0.51 |
Grain Alcohol | 0.23 |
Aluminum | 0.22 |
Copper | 0.09 |
Silver | 0.06 |
Water is therefore one of the most difficult liquids to heat or cool; it can absorb large amounts of heat without increasing its temperature. Remember that temperature reflects the average kinetic energy of the molecules within a substance; the more vigorous the motion, the higher the temperature. In water, the molecules are held together by hydrogen bonds, and these bonds must be overcome to allow the molecules to move freely. When heat is added to water the energy must first go to breaking the hydrogen bonds before the temperature can begin to rise. Therefore, much of the added heat is absorbed by breaking H bonds, not by increasing the temperature, giving water a high heat capacity.
Hydrogen bonds also give water a high latent heat; the heat required to undergo a phase change from solid to liquid, or liquid to gas. The latent heat of fusion is the heat required to go from solid to liquid; 80 cal/g in the case of ice melting to water. Ice is a solid because hydrogen bonds hold the water molecules into a solid crystal lattice (see below). As ice is heated, the temperature rises up to 0o C. At that point, any additional heat goes to melting the ice by breaking the hydrogen bonds, not to increasing the temperature. So as long as ice is present, the water temperature will not increase. This is why your drink will remain cold as long as it contains ice; any heat absorbed goes to melting the ice, not to warming the drink.
When all of the ice is melted, additional heat will increase the temperature of the water 1o C for each calorie of heat added, until it reaches 100o C. At that point, any additional heat goes to overcoming the hydrogen bonds and turning the liquid water into water vapor, rather than increasing the water temperature. The heat required to evaporate liquid water into water vapor is the latent heat of vaporization which has a value of 540 cal/g (Figure 5.1.2).
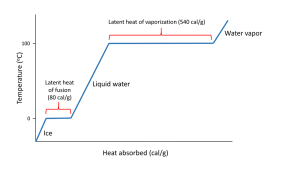
The high heat capacity of water helps regulate global climate, as the oceans slowly absorb and release heat, preventing rapid swings in temperature (see section 8.1). It also means that aquatic organisms aren't as subjected to the same rapid temperature changes as terrestrial organisms. A deep ocean organism may not experience more than a 0.5o C change in temperature over its entire life, while a terrestrial species may encounter changes of more than 20o C in a single day!
3. Water dissolves more substances than any other liquid; it is a "universal solvent", which is why so many substances are dissolved in the ocean. Water is especially good at dissolving ionic salts; molecules made from oppositely charged ions such as NaCl (Na+ and Cl-). In water, the charged ions attract the polar water molecules. The ions get surrounded by a layer of water molecules, weakening the bond between the ions by up to 80 times. With the bonds weakened between ions, the substance dissolves (Figure 5.1.3).
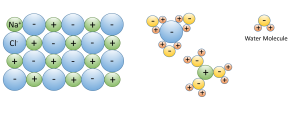
4. The solid phase is less dense than the liquid phase. In other words, ice floats. Most substances are denser in the solid form than in the liquid form, as their molecules are more closely packed together as a solid. Water is an exception: the density of fresh water is 1.0 g/cm3, while the density of ice is 0.92 g/cm3, and once again, this is due to the action of hydrogen bonds.
As water temperature cools the molecules slow down, eventually slowing enough that hydrogen bonds can form and hold the water molecules in a crystal lattice. The molecules in the lattice are spaced farther apart than the molecules in liquid water, which makes ice less dense than liquid water (Figure 5.1.4). This is familiar to anyone who has ever left a full water bottle in the freezer, only to have it burst as the water freezes and expands.
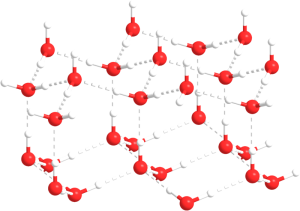
But the relationship between temperature and water density is not a simple linear one. As water cools, its density increases as expected, as the water molecules slow down and get closer together. However, fresh water reaches its maximum density at a temperature of 4o C, and as it cools beyond that point its density declines as the hydrogen bonds begin to form and the intermolecular spacing increases (Figure 5.1.5 inset). The density continues to decline until the temperature reaches 0o C and ice crystals form, reducing the density dramatically (Figure 5.1.5).
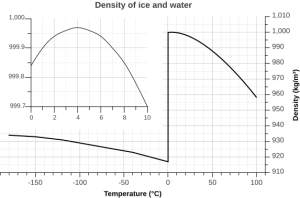
There are a number of important implications to ice being less dense than water. Ice floating on the surface of the ocean helps regulate ocean temperatures, and therefore global climate, by influencing the amount of sunlight that is reflected rather than absorbed (see section 5.6). On a smaller scale, surface ice can prevent lakes and ponds from freezing solid during the winter. As fresh surface water cools, the water gets denser, and sinks to the bottom. The new surface water then cools and sinks, and the process is repeated in what is referred to as overturning, with denser water sinking and less dense water moving to the surface only to be cooled and sink itself. In this way, the entire body of water is cooled somewhat evenly. This process continues until the surface water cools below 4o C. Below 4o C, the water becomes less dense as it cools, so it no longer sinks. Instead, it remains as the surface, getting colder and less dense, until it freezes at 0o C. Once fresh water freezes, the ice floats and insulates the rest of the water beneath it, reducing further cooling. The densest bottom water is still at 4o C, so it does not freeze, allowing the bottom of a lake or pond to remain unfrozen (which is good news for the animals living there) no matter how cold it gets outside.
The dissolved salts in seawater inhibit the formation of the crystal lattice, and therefore make it harder for ice to form. So seawater has a freezing point of about -2o C (depending on salinity), and freezes before a temperature of maximum density is reached. Thus seawater will continue to sink as it gets colder, until it finally freezes.
5. Water has a very high surface tension, the highest of any liquid except mercury (Table 5.1.2). Water molecules are attracted to each other by hydrogen bonds. For molecules not at the water surface, they are surrounded by other water molecules in all directions, so the attractive forces are evenly distributed in all directions. But for molecules at the surface there are few adjacent molecules above them, only below, so all of the attractive forces are directed inwards, away from the surface (Figure 5.1.6). This inwards force is what causes water droplets to take on a spherical shape, and water to bead up on a surface, as the spherical shape provides the minimum possible surface area. These attractive forces also cause the surface of the water to act like an elastic "skin" which allows things like insects to sit on the water's surface without sinking.

Table 5.1.2 Surface tensions of various liquids
Liquid | Surface Tension (millinewton/meter) | Temperature oC |
---|---|---|
Mercury | 487.00 | 15 |
Water | 71.97 | 25 |
Glycerol | 63.00 | 20 |
Acetone | 23.70 | 20 |
Ethanol | 22.27 | 20 |
By Paul Webb, used under a CC-BY 4.0 international license. Download this book for free at https://rwu.pressbooks.pub/webboceanography/front-matter/preface/