40 5.9 Light
Radiant energy from the sun is important for several major oceanic processes:
- Climate, winds, and major ocean currents are ultimately dependent on solar radiation reaching the Earth and heating different areas to different degrees.
- Sunlight warms the surface water where much oceanic life lives.
- Solar radiation provides light for photosynthesis, which supports the entire ocean ecosystem.
The energy reaching Earth from the sun is a form of electromagnetic radiation, which is represented by the electromagnetic spectrum (Figure 5.9.1). Electromagnetic waves vary in their frequency and wavelength. High frequency waves have very short wavelengths, and are very high energy forms of radiation, such as gamma rays and x-rays. These rays can easily penetrate the bodies of living organisms and interfere with individual atoms and molecules. At the other end of the spectrum are low energy, long wavelength waves such as radio waves, which do not pose a hazard to living organisms.
Most of the solar energy reaching the Earth is in the range of visible light, with wavelengths between about 400-700 nm. Each color of visible light has a unique wavelength, and together they make up white light. The shortest wavelengths are on the violet and ultraviolet end of the spectrum, while the longest wavelengths are at the red and infrared end. In between, the colors of the visible spectrum comprise the familiar “ROYGBIV”; red, orange, yellow, green, blue, indigo, and violet.
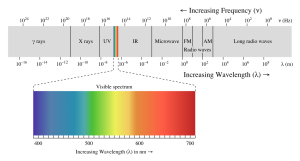
Water is very effective at absorbing incoming light, so the amount of light penetrating the ocean declines rapidly (is attenuated) with depth (Figure 5.9.2). At 1 m depth, only 45% of the solar energy that falls on the ocean surface remains. At 10 m depth only 16% of the light is still present, and only 1% of the original light is left at 100 m. No light penetrates beyond 1000 m.
In addition to overall attenuation, the oceans absorb the different wavelengths of light at different rates (Figure 5.9.2). The wavelengths at the extreme ends of the visible spectrum are attenuated faster than those wavelengths in the middle. Longer wavelengths are absorbed first; red is absorbed in the upper 10 m, orange by about 40 m, and yellow disappears before 100 m. Shorter wavelengths penetrate further, with blue and green light reaching the deepest depths.
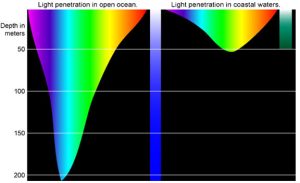
This explains why everything appears blue under water. The colors we perceive depends on the wavelengths of light that are received by our eyes. If an object appears red to us, that is because the object reflects red light but absorbs all of the other colors. So the only color reaching our eyes is red. Under water, blue is the only color of light still available at depth, so that is the only color that can be reflected back to our eyes, and everything has a blue tinge under water. A red object at depth will not appear red to us because there is no red light available to reflect off of the object. Objects in water will only appear as their real colors near the surface where all wavelengths of light are still available, or if the other wavelengths of light are provided artificially, such as by illuminating the object with a dive light.
Water in the open ocean appears clear and blue because it contains much less particulate matter, such as phytoplankton or other suspended particles, and the clearer the water, the deeper the light penetration. Blue light penetrates deeply and is scattered by the water molecules, while all other colors are absorbed; thus the water appears blue. On the other hand, coastal water often appears greenish (Figure 5.9.2). Coastal water contains much more suspended silt and algae and microscopic organisms than the open ocean. Many of these organisms, such as phytoplankton, absorb light in the blue and red range through their photosynthetic pigments, leaving green as the dominant wavelength of reflected light. Therefore the higher the phytoplankton concentration in water, the greener it appears. Small silt particles may also absorb blue light, further shifting the color of water away from blue when there are high concentrations of suspended particles.
The ocean can be divided into depth layers depending on the amount of light penetration, as discussed in section 1.3 (Figure 5.9.3). The upper 200 m is referred to as the photic or euphotic zone. This represents the region where enough light can penetrate to support photosynthesis, and it corresponds to the epipelagic zone. From 200-1000 m lies the dysphotic zone, or the twilight zone (corresponding with the mesopelagic zone). There is still some light at these depths, but not enough to support photosynthesis. Below 1000 m is the aphotic (or midnight) zone, where no light penetrates. This region includes the majority of the ocean volume, which exists in complete darkness.
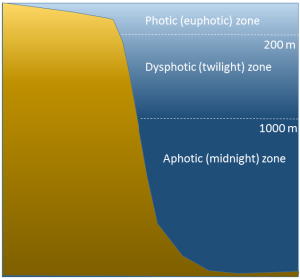
Estuaries are partially enclosed bodies of water where the salt water is diluted by fresh water input from land, creating brackish water with a salinity somewhere between fresh water and normal seawater. Estuaries include many bays, inlets, and sounds, and are often subject to large temperature and salinity variations due to their enclosed nature and smaller size compared to the open ocean.
Estuaries can be classified geologically into four basic categories based on their method of origin. In all cases they are a result of rising sea level over the last 18,000 years, beginning with the end of the last ice age; a period that has seen a rise of about 130 m. The rise in sea level has flooded coastal areas that were previously above water, and prevented the estuaries from being filled in by all of the sediments that have been emptied into them.
The first type is a coastal plain estuary, or drowned river valley. These estuaries are formed as sea level rises and floods an existing river valley, mixing salt and fresh water to create the brackish conditions where the river meets the sea. These types of estuaries are common along the east coast of the United States, including major bodies such as the Chesapeake Bay, Delaware Bay, and Narragansett Bay (Figure 4.6.1). Coastal plain estuaries are usually shallow, and since there is a lot of sediment input from the rivers, there are often a number of depositional features associated with them such as spits and barrier islands.
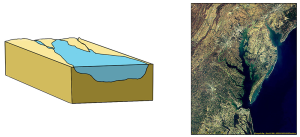
The presence of sand bars, spits, and barrier islands can lead to bar-built estuaries, where a barrier is created between the mainland and the ocean. The water that remains inside the sand bar is cut off from complete mixing with the ocean, and receives freshwater input from the mainland, creating estuarine conditions (Figure 4.6.2).
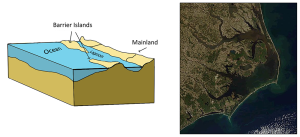
Fjords are estuaries formed in deep, U-shaped basins that were carved out by advancing glaciers. When the glaciers melted and retreated, sea level rose and filled these troughs, creating deep, steep-walled fjords (Figure 4.6.3). Fjords are common in Norway, Alaska, Canada, and New Zealand, where there are mountainous coastlines once covered by glaciers.
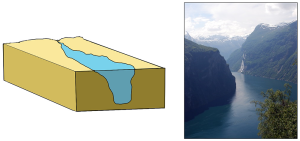
Tectonic estuaries are the result of tectonic movements, where faulting causes some sections of the crust to subside, and those lower elevation sections then get flooded with seawater. San Francisco Bay is an example of a tectonic estuary (Figure 4.6.4).
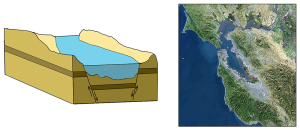
Estuaries are also classified based on their salinity and mixing patterns. The amount of mixing of fresh and salt water in an estuary depends on the rate at which fresh water enters the head of the estuary from river input, and the amount of seawater that enters the estuary mouth as a result of tidal movements. The input of fresh water is reflected in the flushing time of the estuary. This refers to the time it would take for the in-flowing fresh water to completely replace all the fresh water currently in the estuary. Seawater input is measured by the tidal volume, or tidal prism, which is the average volume of sea water entering and leaving the estuary during each tidal cycle. In other words, it is the volume difference between high and low tides. The interaction between the flushing time, tidal volume, and the shape of the estuary will determine the extent and type of water mixing within the estuary.
In a vertically mixed, or well-mixed estuary there is complete mixing of fresh and salt water from the surface to the bottom. In a particular location the salinity is constant at all depths, but across the estuary the salinity is lowest at the head where the fresh water enters, and is highest at the mouth, where the seawater comes in. This type of salinity profile usually occurs in shallower estuaries, where the shallow depths allow complete mixing from the surface to the bottom.
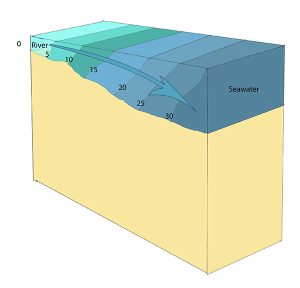
Slightly stratified or partially mixed estuaries have similar salinity profiles to vertically mixed estuaries, where salinity increases from the head to the mouth, but there is also a slight increase in salinity with depth at any point. This usually occurs in deeper estuaries than those that are well-mixed, where waves and currents mix the surface water, but the mixing may not extend all the way to the bottom.
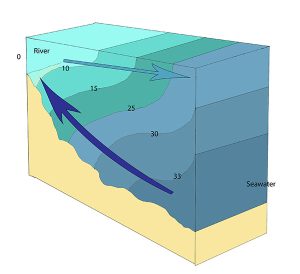
A salt wedge estuary occurs where the outflow of fresh water is strong enough to prevent the denser ocean water to enter through the surface, and where the estuary is deep enough that surface waves and turbulence have little mixing effect on the deeper water. Fresh water flows out along surface, salt water flows in at depth, creating a wedge shaped lens of seawater moving along the bottom. The surface water may remain mostly fresh throughout the estuary if there is no mixing, or it can become brackish depending on the level of mixing that occurs.

Highly stratified profiles are found in very deep estuaries, such as in fjords. Because of the depth, mixing of fresh and salt water only occurs near the surface, so in the upper layers salinity increases from the head to the mouth, but the deeper water is of standard ocean salinity.

Estuaries are very important commercially, as they are home to the majority of the world’s metropolitan areas, they serve as ports for industrial activity, and a large percentage of the world's population lives near estuaries. Estuaries are also very important biologically, especially in their role as the breeding grounds for many species of fish, birds, and invertebrates.
By Paul Webb, used under a CC-BY 4.0 international license. Download this book for free at https://rwu.pressbooks.pub/webboceanography/front-matter/preface/
Estuaries are partially enclosed bodies of water where the salt water is diluted by fresh water input from land, creating brackish water with a salinity somewhere between fresh water and normal seawater. Estuaries include many bays, inlets, and sounds, and are often subject to large temperature and salinity variations due to their enclosed nature and smaller size compared to the open ocean.
Estuaries can be classified geologically into four basic categories based on their method of origin. In all cases they are a result of rising sea level over the last 18,000 years, beginning with the end of the last ice age; a period that has seen a rise of about 130 m. The rise in sea level has flooded coastal areas that were previously above water, and prevented the estuaries from being filled in by all of the sediments that have been emptied into them.
The first type is a coastal plain estuary, or drowned river valley. These estuaries are formed as sea level rises and floods an existing river valley, mixing salt and fresh water to create the brackish conditions where the river meets the sea. These types of estuaries are common along the east coast of the United States, including major bodies such as the Chesapeake Bay, Delaware Bay, and Narragansett Bay (Figure 4.6.1). Coastal plain estuaries are usually shallow, and since there is a lot of sediment input from the rivers, there are often a number of depositional features associated with them such as spits and barrier islands.
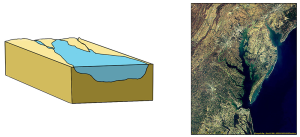
The presence of sand bars, spits, and barrier islands can lead to bar-built estuaries, where a barrier is created between the mainland and the ocean. The water that remains inside the sand bar is cut off from complete mixing with the ocean, and receives freshwater input from the mainland, creating estuarine conditions (Figure 4.6.2).
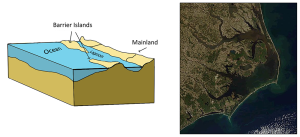
Fjords are estuaries formed in deep, U-shaped basins that were carved out by advancing glaciers. When the glaciers melted and retreated, sea level rose and filled these troughs, creating deep, steep-walled fjords (Figure 4.6.3). Fjords are common in Norway, Alaska, Canada, and New Zealand, where there are mountainous coastlines once covered by glaciers.
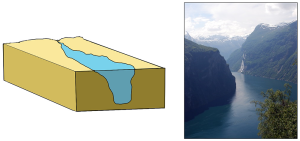
Tectonic estuaries are the result of tectonic movements, where faulting causes some sections of the crust to subside, and those lower elevation sections then get flooded with seawater. San Francisco Bay is an example of a tectonic estuary (Figure 4.6.4).
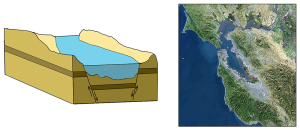
Estuaries are also classified based on their salinity and mixing patterns. The amount of mixing of fresh and salt water in an estuary depends on the rate at which fresh water enters the head of the estuary from river input, and the amount of seawater that enters the estuary mouth as a result of tidal movements. The input of fresh water is reflected in the flushing time of the estuary. This refers to the time it would take for the in-flowing fresh water to completely replace all the fresh water currently in the estuary. Seawater input is measured by the tidal volume, or tidal prism, which is the average volume of sea water entering and leaving the estuary during each tidal cycle. In other words, it is the volume difference between high and low tides. The interaction between the flushing time, tidal volume, and the shape of the estuary will determine the extent and type of water mixing within the estuary.
In a vertically mixed, or well-mixed estuary there is complete mixing of fresh and salt water from the surface to the bottom. In a particular location the salinity is constant at all depths, but across the estuary the salinity is lowest at the head where the fresh water enters, and is highest at the mouth, where the seawater comes in. This type of salinity profile usually occurs in shallower estuaries, where the shallow depths allow complete mixing from the surface to the bottom.
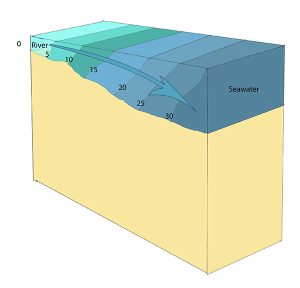
Slightly stratified or partially mixed estuaries have similar salinity profiles to vertically mixed estuaries, where salinity increases from the head to the mouth, but there is also a slight increase in salinity with depth at any point. This usually occurs in deeper estuaries than those that are well-mixed, where waves and currents mix the surface water, but the mixing may not extend all the way to the bottom.
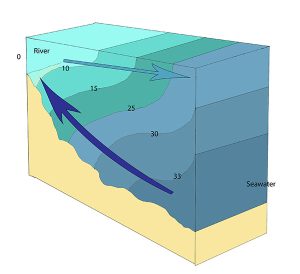
A salt wedge estuary occurs where the outflow of fresh water is strong enough to prevent the denser ocean water to enter through the surface, and where the estuary is deep enough that surface waves and turbulence have little mixing effect on the deeper water. Fresh water flows out along surface, salt water flows in at depth, creating a wedge shaped lens of seawater moving along the bottom. The surface water may remain mostly fresh throughout the estuary if there is no mixing, or it can become brackish depending on the level of mixing that occurs.
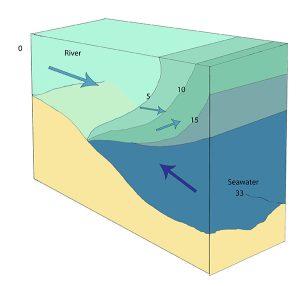
Highly stratified profiles are found in very deep estuaries, such as in fjords. Because of the depth, mixing of fresh and salt water only occurs near the surface, so in the upper layers salinity increases from the head to the mouth, but the deeper water is of standard ocean salinity.

Estuaries are very important commercially, as they are home to the majority of the world’s metropolitan areas, they serve as ports for industrial activity, and a large percentage of the world's population lives near estuaries. Estuaries are also very important biologically, especially in their role as the breeding grounds for many species of fish, birds, and invertebrates.
By Paul Webb, used under a CC-BY 4.0 international license. Download this book for free at https://rwu.pressbooks.pub/webboceanography/front-matter/preface/
Waves generally begin as a disturbance of some kind, and the energy of that disturbance gets propagated in the form of waves. We are most familiar with the kind of waves that break on shore, or rock a boat at sea, but there are many other types of waves that are important to oceanography:
- Internal waves form at the boundaries of water masses of different densities (i.e. at a pycnocline), and propagate at depth. These generally move more slowly than surface waves, and can be much larger, with heights exceeding 100 m. However, the height of the deep wave would be unnoticeable at the surface.
- Tidal waves are due to the movement of the tides. What we think of as tides are basically enormously long waves with a wavelength that may span half the globe (see section 4.1). Tidal waves are not related to tsunamis, so don’t confuse the two.
- Tsunamis are large waves created as a result of earthquakes or other seismic disturbances. They are also called seismic sea waves (section 3.4).
- Splash waves are formed when something falls into the ocean and creates a splash. The giant wave in Lituya Bay that was described in the introduction to this chapter was a splash wave.
- Atmospheric waves form in the sky at the boundary between air masses of different densities. These often create ripple effects in the clouds (Figure 3.1.1).
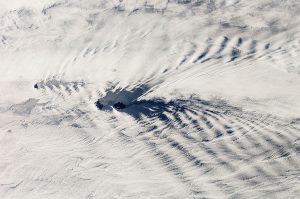
There are several components to a basic wave (Figure 3.1.2):
- Still water level: where the water surface would be if there were no waves present and the sea was completely calm.
- Crest: the highest point of the wave.
- Trough: the lowest point of the wave.
- Wave height: the distance between the crest and the trough.
- Wavelength: the distance between two identical points on successive waves, for example crest to crest, or trough to trough.
- Wave steepness: the ratio of wave height to length (H/L). If this ratio exceeds 1/7 (i.e. height exceeds 1/7 of the wavelength) the wave gets too steep, and will break.
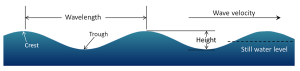
There are also a number of terms used to describe wave motion:
- Period: the time it takes for two successive crests to pass a given point.
- Frequency: the number of waves passing a point in a given amount of time, usually expressed as waves per second. This is the inverse of the period.
- Speed: how fast the wave travels, or the distance traveled per unit of time. This is also called celerity (c), where
c = wavelength x frequency
Therefore, the longer the wavelength, the faster the wave.
Although waves can travel over great distances, the water itself shows little horizontal movement; it is the energy of the wave that is being transmitted, not the water. Instead, the water particles move in circular orbits, with the size of the orbit equal to the wave height (Figure 3.1.3). This orbital motion occurs because water waves contain components of both longitudinal (side to side) and transverse (up and down) waves, leading to circular motion. As a wave passes, water moves forwards and up over the wave crests, then down and backwards into the troughs, so there is little horizontal movement. This is evident if you have ever watched an object such as a seabird floating at the surface. The bird bobs up and down as the wave pass underneath it; it does not get carried horizontally by a single wave crest.
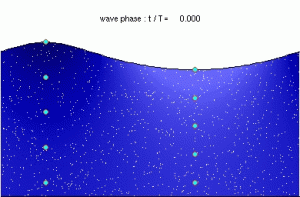
The circular orbital motion declines with depth as the wave has less impact on deeper water and the diameter of the circles is reduced. Eventually at some depth there is no more circular movement and the water is unaffected by surface wave action. This depth is the wave base and is equivalent to half of the wavelength (Figure 3.1.4). Since most ocean waves have wavelengths of less than a few hundred meters, most of the deeper ocean is unaffected by surface waves, so even in the strongest storms marine life or submarines can avoid heavy waves by submerging below the wave base.

When the water below a wave is deeper than the wave base (deeper than half of the wavelength), those waves are called deep water waves. Most open ocean waves are deep water waves. Since the water is deeper than the wave base, deep water waves experience no interference from the bottom, so their speed only depends on the wavelength:
[latex]\text{speed (m/s)} = \sqrt{\frac{gL}{2\pi}}[/latex]
where g is gravity and L is wavelength in meters. Since g and π are constants, this can be simplified to:
[latex]\text{speed (m/s)} = 1.25\sqrt{L}[/latex]
Shallow water waves occur when the depth is less than 1/20 of the wavelength. In these cases, the wave is said to "touch bottom" because the depth is shallower than the wave base so the orbital motion is affected by the seafloor. Due to the shallow depth, the orbits are flattened, and eventually the water movement becomes horizontal rather than circular just above the bottom. The speed of shallow water waves depends only on the depth:
[latex]\text{speed (m/s)} = \sqrt{gd}[/latex]
where g is gravity and d is depth in meters. This can be simplified to:
[latex]\text{speed (m/s)} = 3.13\sqrt{d}[/latex]
Intermediate or transitional waves are found in depths between ½ and 1/20 of the wavelength. Their behavior is a bit more complex, as their speed is influenced by both wavelength and depth. The speed of an intermediate wave is calculated as:
which contains both depth and wavelength variables.
Waves generally begin as a disturbance of some kind, and the energy of that disturbance gets propagated in the form of waves. We are most familiar with the kind of waves that break on shore, or rock a boat at sea, but there are many other types of waves that are important to oceanography:
- Internal waves form at the boundaries of water masses of different densities (i.e. at a pycnocline), and propagate at depth. These generally move more slowly than surface waves, and can be much larger, with heights exceeding 100 m. However, the height of the deep wave would be unnoticeable at the surface.
- Tidal waves are due to the movement of the tides. What we think of as tides are basically enormously long waves with a wavelength that may span half the globe (see section 4.1). Tidal waves are not related to tsunamis, so don’t confuse the two.
- Tsunamis are large waves created as a result of earthquakes or other seismic disturbances. They are also called seismic sea waves (section 3.4).
- Splash waves are formed when something falls into the ocean and creates a splash. The giant wave in Lituya Bay that was described in the introduction to this chapter was a splash wave.
- Atmospheric waves form in the sky at the boundary between air masses of different densities. These often create ripple effects in the clouds (Figure 3.1.1).
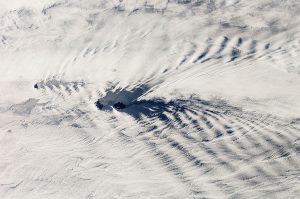
There are several components to a basic wave (Figure 3.1.2):
- Still water level: where the water surface would be if there were no waves present and the sea was completely calm.
- Crest: the highest point of the wave.
- Trough: the lowest point of the wave.
- Wave height: the distance between the crest and the trough.
- Wavelength: the distance between two identical points on successive waves, for example crest to crest, or trough to trough.
- Wave steepness: the ratio of wave height to length (H/L). If this ratio exceeds 1/7 (i.e. height exceeds 1/7 of the wavelength) the wave gets too steep, and will break.
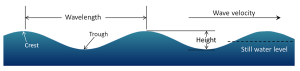
There are also a number of terms used to describe wave motion:
- Period: the time it takes for two successive crests to pass a given point.
- Frequency: the number of waves passing a point in a given amount of time, usually expressed as waves per second. This is the inverse of the period.
- Speed: how fast the wave travels, or the distance traveled per unit of time. This is also called celerity (c), where
c = wavelength x frequency
Therefore, the longer the wavelength, the faster the wave.
Although waves can travel over great distances, the water itself shows little horizontal movement; it is the energy of the wave that is being transmitted, not the water. Instead, the water particles move in circular orbits, with the size of the orbit equal to the wave height (Figure 3.1.3). This orbital motion occurs because water waves contain components of both longitudinal (side to side) and transverse (up and down) waves, leading to circular motion. As a wave passes, water moves forwards and up over the wave crests, then down and backwards into the troughs, so there is little horizontal movement. This is evident if you have ever watched an object such as a seabird floating at the surface. The bird bobs up and down as the wave pass underneath it; it does not get carried horizontally by a single wave crest.
Figure 3.1.3 Animation showing the orbital motion of particles in a surface wave (By Kraaiennest (Own work) [GFDL (http://www.gnu.org/copyleft/fdl.html) or CC BY-SA 4.0], via Wikimedia Commons).
The circular orbital motion declines with depth as the wave has less impact on deeper water and the diameter of the circles is reduced. Eventually at some depth there is no more circular movement and the water is unaffected by surface wave action. This depth is the wave base and is equivalent to half of the wavelength (Figure 3.1.4). Since most ocean waves have wavelengths of less than a few hundred meters, most of the deeper ocean is unaffected by surface waves, so even in the strongest storms marine life or submarines can avoid heavy waves by submerging below the wave base.
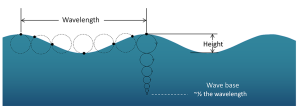
When the water below a wave is deeper than the wave base (deeper than half of the wavelength), those waves are called deep water waves. Most open ocean waves are deep water waves. Since the water is deeper than the wave base, deep water waves experience no interference from the bottom, so their speed only depends on the wavelength:
[latex]\text{speed (m/s)} = \sqrt{\frac{gL}{2\pi}}[/latex]
where g is gravity and L is wavelength in meters. Since g and π are constants, this can be simplified to:
[latex]\text{speed (m/s)} = 1.25\sqrt{L}[/latex]
Shallow water waves occur when the depth is less than 1/20 of the wavelength. In these cases, the wave is said to "touch bottom" because the depth is shallower than the wave base so the orbital motion is affected by the seafloor. Due to the shallow depth, the orbits are flattened, and eventually the water movement becomes horizontal rather than circular just above the bottom. The speed of shallow water waves depends only on the depth:
[latex]\text{speed (m/s)} = \sqrt{gd}[/latex]
where g is gravity and d is depth in meters. This can be simplified to:
[latex]\text{speed (m/s)} = 3.13\sqrt{d}[/latex]
Intermediate or transitional waves are found in depths between ½ and 1/20 of the wavelength. Their behavior is a bit more complex, as their speed is influenced by both wavelength and depth. The speed of an intermediate wave is calculated as:
which contains both depth and wavelength variables.
Waves generally begin as a disturbance of some kind, and the energy of that disturbance gets propagated in the form of waves. We are most familiar with the kind of waves that break on shore, or rock a boat at sea, but there are many other types of waves that are important to oceanography:
- Internal waves form at the boundaries of water masses of different densities (i.e. at a pycnocline), and propagate at depth. These generally move more slowly than surface waves, and can be much larger, with heights exceeding 100 m. However, the height of the deep wave would be unnoticeable at the surface.
- Tidal waves are due to the movement of the tides. What we think of as tides are basically enormously long waves with a wavelength that may span half the globe (see section 4.1). Tidal waves are not related to tsunamis, so don’t confuse the two.
- Tsunamis are large waves created as a result of earthquakes or other seismic disturbances. They are also called seismic sea waves (section 3.4).
- Splash waves are formed when something falls into the ocean and creates a splash. The giant wave in Lituya Bay that was described in the introduction to this chapter was a splash wave.
- Atmospheric waves form in the sky at the boundary between air masses of different densities. These often create ripple effects in the clouds (Figure 3.1.1).
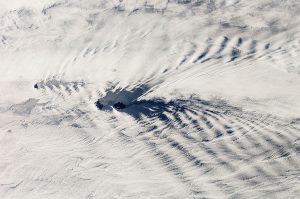
There are several components to a basic wave (Figure 3.1.2):
- Still water level: where the water surface would be if there were no waves present and the sea was completely calm.
- Crest: the highest point of the wave.
- Trough: the lowest point of the wave.
- Wave height: the distance between the crest and the trough.
- Wavelength: the distance between two identical points on successive waves, for example crest to crest, or trough to trough.
- Wave steepness: the ratio of wave height to length (H/L). If this ratio exceeds 1/7 (i.e. height exceeds 1/7 of the wavelength) the wave gets too steep, and will break.
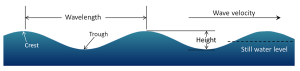
There are also a number of terms used to describe wave motion:
- Period: the time it takes for two successive crests to pass a given point.
- Frequency: the number of waves passing a point in a given amount of time, usually expressed as waves per second. This is the inverse of the period.
- Speed: how fast the wave travels, or the distance traveled per unit of time. This is also called celerity (c), where
c = wavelength x frequency
Therefore, the longer the wavelength, the faster the wave.
Although waves can travel over great distances, the water itself shows little horizontal movement; it is the energy of the wave that is being transmitted, not the water. Instead, the water particles move in circular orbits, with the size of the orbit equal to the wave height (Figure 3.1.3). This orbital motion occurs because water waves contain components of both longitudinal (side to side) and transverse (up and down) waves, leading to circular motion. As a wave passes, water moves forwards and up over the wave crests, then down and backwards into the troughs, so there is little horizontal movement. This is evident if you have ever watched an object such as a seabird floating at the surface. The bird bobs up and down as the wave pass underneath it; it does not get carried horizontally by a single wave crest.

The circular orbital motion declines with depth as the wave has less impact on deeper water and the diameter of the circles is reduced. Eventually at some depth there is no more circular movement and the water is unaffected by surface wave action. This depth is the wave base and is equivalent to half of the wavelength (Figure 3.1.4). Since most ocean waves have wavelengths of less than a few hundred meters, most of the deeper ocean is unaffected by surface waves, so even in the strongest storms marine life or submarines can avoid heavy waves by submerging below the wave base.

When the water below a wave is deeper than the wave base (deeper than half of the wavelength), those waves are called deep water waves. Most open ocean waves are deep water waves. Since the water is deeper than the wave base, deep water waves experience no interference from the bottom, so their speed only depends on the wavelength:
[latex]\text{speed (m/s)} = \sqrt{\frac{gL}{2\pi}}[/latex]
where g is gravity and L is wavelength in meters. Since g and π are constants, this can be simplified to:
[latex]\text{speed (m/s)} = 1.25\sqrt{L}[/latex]
Shallow water waves occur when the depth is less than 1/20 of the wavelength. In these cases, the wave is said to "touch bottom" because the depth is shallower than the wave base so the orbital motion is affected by the seafloor. Due to the shallow depth, the orbits are flattened, and eventually the water movement becomes horizontal rather than circular just above the bottom. The speed of shallow water waves depends only on the depth:
[latex]\text{speed (m/s)} = \sqrt{gd}[/latex]
where g is gravity and d is depth in meters. This can be simplified to:
[latex]\text{speed (m/s)} = 3.13\sqrt{d}[/latex]
Intermediate or transitional waves are found in depths between ½ and 1/20 of the wavelength. Their behavior is a bit more complex, as their speed is influenced by both wavelength and depth. The speed of an intermediate wave is calculated as:
which contains both depth and wavelength variables.
It may seem odd to be discussing coral reefs in a section about geology, but due to the stony calcium carbonate skeletons secreted by many coral species, coral reefs are as interesting as geological features as they are biological ones. Corals grow best in warm, clear, tropical water, that is close enough to the surface for light to support photosynthesis by the algae living in the coral tissues. Because of this need for light, new coral will often grown on top of the stony skeletons of older corals.
In the 1830s Charles Darwin made some observations about different types of coral reefs, and hypothesized that they represent a progression from one form to the next. The types of reefs he examined were fringing reefs, barrier reefs, and atolls, which are associated with oceanic islands (Figure 2.10.1). Fringing reefs are reefs that are close to or are connected to shore. Barrier reefs are offshore reefs that are separated from the land by an expanse of water, such as a lagoon. Atolls are circular or oval reefs surrounding a lagoon, without any central land mass in the lagoon. Darwin speculated that reefs progressed from fringing, to barrier, to atolls as the land mass subsided. However, he had no explanation for how volcanic islands could sink. Today we know that Darwin was correct, and that islands can sink as oceanic crust subsides as it moves away from a spreading center, or as sea level rises as glaciers melt.
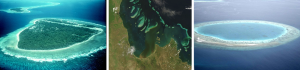

Large waves crashing onto a shore bring a tremendous amount of energy that has a significant eroding effect, and several unique erosion features commonly form on rocky shores with strong waves.
When waves approach an irregular shore, they are slowed down to varying degrees, depending on differences in the water depth, and as they slow, they are bent or refracted (section 3.3). In Figure 4.3.1, wave energy is represented by the blue arrows. That energy is evenly spaced out in the deep water, but because of refraction, the energy of the waves is being focused on the headlands. On irregular coasts, the headlands receive much more wave energy than the intervening bays, and thus they are more strongly eroded. The result of this is coastal straightening, where an irregular coast will eventually become straightened, although that process may take millions of years.
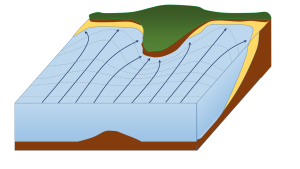
Wave erosion is greatest in the surf zone, where the wave base is impinging strongly on the seafloor and where the waves are breaking. The result is that the substrate in the surf zone is typically eroded to a flat surface known as a wave-cut platform (or wave-cut terrace) (Figure 4.3.2). A wave-cut platform extends across the intertidal zone.

Arches and sea caves form as a result of the erosion of relatively non-resistant rock. Wave action and strong longshore currents can carve a cave into a headland, and if the erosion extends all the way through, it becomes an arch. If a hole develops in the ceiling of a cave, a blowhole can be created, shooting water into the air when waves crash in the cave. An arch in the Barachois River area of western Newfoundland, Canada, is shown in Figure 4.3.3. This feature started out as a sea cave, and then, after being eroded from both sides, became an arch. During the winter of 2012-2013, the arch collapsed, leaving a small stack at the end of the point.

The tower of rock left behind from a collapsed arch is called a sea stack (Figure 4.3.4). But sea stacks can also form during the formation of wave-cut platforms or other features, when relatively resistant rock that does not get completely eroded remains behind to form the stack.


By Paul Webb, used under a CC-BY 4.0 international license. Download this book for free at https://rwu.pressbooks.pub/webboceanography/front-matter/preface/
Modified from "Physical Geology" by Steven Earle used under a CC-BY 4.0 international license. Download this book for free at http://open.bccampus.ca
Continental margins refer to the region of transition from the land to the deep seafloor, i.e. between continental and oceanic crust. In an active continental margin, the boundary between the continent and the ocean is also a tectonic plate boundary, so there is a lot of geological activity around the margin. The west coast of the United States is an example of an active margin, where the coastline corresponds with the boundary between the Pacific and North America Plates. A passive continental margin occurs where the transition from land to sea is not associated with a plate boundary. The east coast of the United States is a good example; the plate boundary is located along the mid Atlantic ridge, far from the coast. Passive margins are less geologically active. Figure 1.2.1 shows an idealized passive margin. When examining this figure, and others like it, note that there is significant vertical exaggeration; the depth scale covers approximately 5000 m, while the horizontal scale extends around 300 km. This makes the features look much steeper than they actually are. The bar at the bottom of Figure 1.2.1 shows what a passive margin would look like without this exaggeration; there is a much more gradual transition to depth.
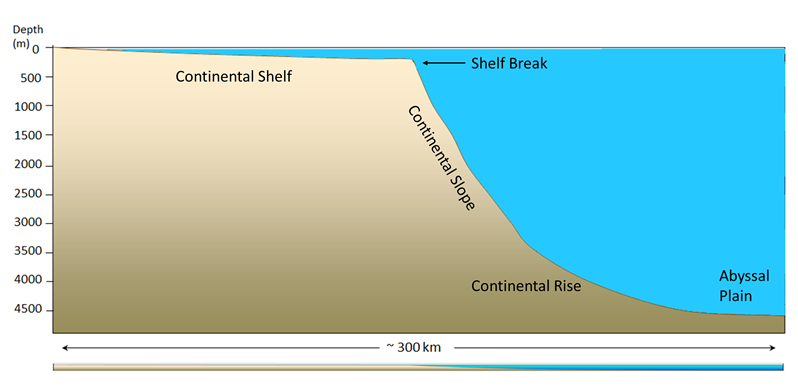
The continental shelf is the shallow, flooded edge of the continent. Geologically the shelf is still part of the continental crust, but it is often overlaid with marine sediments. On average, the shelf extends about 80 km from the coast; some margins have very little shelf, while the Siberian Shelf in the Arctic extends roughly 1500 km. The depth of the shelf generally remains below about 150 m, and the floor of the shelf is fairly flat. The flat topography is the result of changes in sea level; throughout history the shelves have been both submerged and exposed, and as sea level rose and fell, wave action, ice sheets, and other erosional processes smoothed out the shelf surface. Wave action and the movement of sediments over the shelf have continued this smoothing process. Continental shelves only make up about 6% of the ocean's surface area, but they are biologically one of the richest parts of the ocean; their shallow depth prevents nutrients from sinking out, and their proximity to the coast provides significant nutrient input. The continental shelf ends at the shelf break, which is the point where the angle of the seafloor begins to get steeper. The shelf break averages about 135 m deep.
After the shelf break, the seafloor takes on a steeper angle (about 4o) as it descends to the deep ocean. This steeper portion of the margin is the continental slope, and it extends from the shelf break down to 3000-5000m. In some parts of the ocean, large submarine canyons have been carved into the continental slope; for example, Monterey Canyon in Monterey Bay, California, is a submarine canyon similar in size to the Grand Canyon! These canyons may be carved out by turbidity currents, which are essentially landslides of sediment, rocks, and other debris down the face of the slope.
At the bottom of the slope is the continental rise. This area represents where the continental crust meets the oceanic crust, as the slope begins to level off to become the deep ocean floor. The rise consists of a thick layer of accumulated sediment coming from the continent, so it is difficult to tell where the slope ends and the rise begins.
After the rise comes the abyssal plain, or the deep ocean floor, lying between 4500 - 6000 m. The abyssal plain includes most of the ocean floor, and is the flattest region on Earth. It is flat due to millions of years of sediment accumulation on the bottom, which buries many bottom features (Figure 1.2.2).
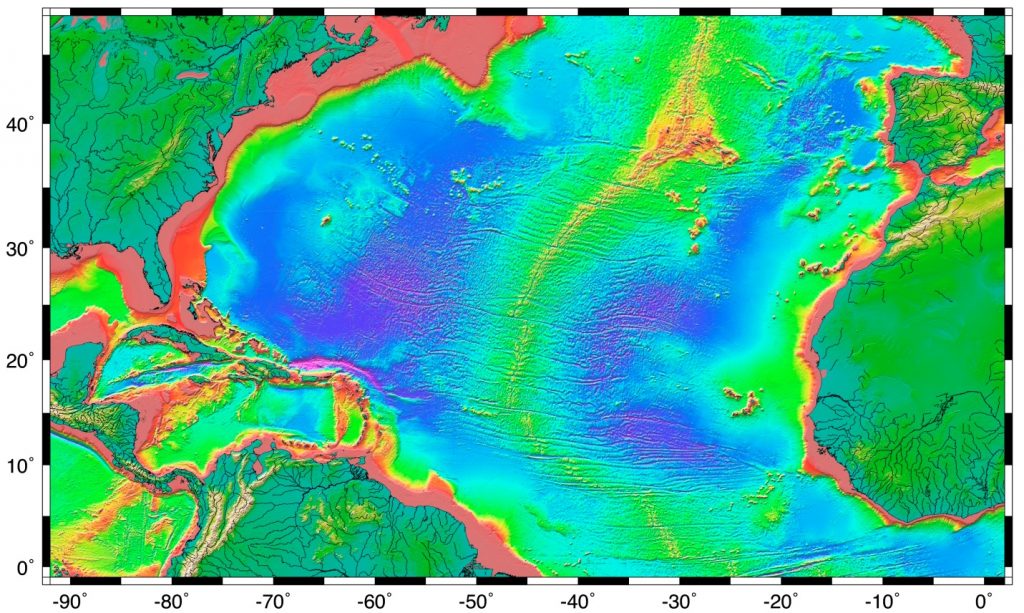
Passive margins, as described above, have wide shelves, gentle slopes, and a well-developed rise. Since passive margins are not plate boundaries, they experience long periods of relative stability which can lead to the development of these features. Active margins have similar features to passive margins, but the plate boundary affects the properties of the features. Active margins, like the Pacific coast of North America, have narrower shelves, steeper slopes, and little to no rise, particularly in convergent boundaries. Trenches associated with subduction zones act as sediment traps, preventing the accumulation of a continental rise, and keeping sediments off of the abyssal plains.